Микология и фитопатология, 2022, T. 56, № 4, стр. 231-244
Organic Acids Production by Fungi: Metabolism, Physiological and Ecological Significance
K. V. Sazanova a, b, *, **, N. G. Osmolovskaya c, ***, D. Yu. Vlasov a, c, ****
a V.L. Komarov Botanical Institute of the Russian Academy of Sciences
197376 St. Petersburg, Russia
b Archive of the Russian Academy of Sciences, St. Petersburg Branch
196084 St. Petersburg, Russia
c Saint Petersburg State University
199034 St. Petersburg, Russia
* E-mail: ksazanova@binran.ru
** E-mail: barinova-kv@mail.ru
*** E-mail: n.osmolovskaya@spbu.ru
**** E-mail: dmitry.vlasov@mail.ru
Поступила в редакцию 15.05.2021
После доработки 21.04.2022
Принята к публикации 7.05.2022
- EDN: PCIICE
- DOI: 10.31857/S0026364822040092
Аннотация
The data about pathways of biosynthesis, regulation of metabolism, physiological and ecological functions of organic acids production by fungi of various ecological groups are generalized and analyzed. Metabolism and excretion of organic acids by fungi depend on many factors, including nutrient and mineral conditions, temperature, and various stresses. An increase in the excretion of organic acids, especially oxalic acid by fungi is often a response to stress. Organic acids production by fungi has important ecological and physiological significance because it is related to changing the surrounding microconditions and influencing the biotic and abiotic interactions of microorganisms in community. Organic acids are involved in the formation of mycorrhiza, the processes of plant pathogenesis, and are also one of the key factors in wood decay. The ability to acidification largely determines the geochemical role of fungi and their importance in the weathering of rocks and the processes of primary soil formation.
INTRODUCTION
Low molecular weight organic acids (LMWOAs) – one of the most important molecules in energy metabolism and regulators of metabolic processes in the cells of living organisms. LMWOAs are of fundamental importance for formation of precursors for amino acid biosynthesis, take part in the biosynthesis of alkaloids, glycosides and other biologically active compounds, and serve as a link between the individual stages of the metabolism of fats, proteins and carbohydrates. In addition LMWOAs involved in the pH regulation, maintaining of ion homeostasis in the cell and its osmotic potential (Sharma et al., 2016; Wang et al., 2019). At the same time, a number of organisms, including plants, bacteria and fungi, are able to excrete synthesized organic acids into environment (López-Bucio, 2000; Ryan et al., 2001; Yadav et al., 2022) while the prevalence and intensity of this phenomenon in fungi is incomparably higher (Magnuson, Lasure, 2004; Papagianni, 2007).
The first information about the production of organic acids by fungi dates back to the second half of the 19th century (Hamlet, Plowright, 1877; Zhuravsky, 1939). Most researchers at the beginning of the 20th century, before establishing the sequence of reactions of the tricarboxylic acid cycle (TCA cycle), assumed the closest relationship between the formation of citric, succinic, malic and fumaric acids with the process of sugar oxidation. The pathways for the biosynthesis of organic acids from carbohydrates were first described by Franzen, Schmitt, Challenger, Walker, and Subramaniam (Bennet-Clark, 1938). Later, it was noted that organic acids formed by fungi first accumulate in the nutrient solution and then disappear. This circumstance gave rise to the idea that acids are used as a source of nutrition when sugars are depleted in the nutrient medium. Those LMWOAs are intermediates in the oxidation of sugars to carbon dioxide (Butkevich, 1957).
At present, the pathways of organic acid metabolism are fairly well understood. There are many works about its regulation, especially great attention is paid to the hyperproduction of acids that are of biotechnological importance. However the specific functions of acids and the reasons for their hyperproduction in fungi are not always understood, and the available data are clearly not sufficient to explain this phenomenon.
Most often in the largest amount gluconic, citric, malic, fumaric, succinic and oxalic acids are produced by fungi (Max et al., 2010; Sazanova et al., 2015, 2016; Show et al., 2015; Almousa et al., 2018). Obviously for fungi organic acids acquire a particularly important ecological and physiological significance, including in view of their changes in the surrounding micro-conditions and influencing biotic and abiotic relationships in the community. In the natural habitat, the production of organic acids has many ecological functions, which are discussed in detail in this review. At the same time, it is very labile process, depended on many factors, and at present there are only a few attempts to extrapolate the data obtained in vitro to the processes in nature.
The production of organic acids by fungi is of great importance for applied mycology and biotechnology. For industrial purposes fungal strains are used that are characterized by overproduction of organic acids when cultivated on artificially selected media. According to some data, Aspergillus niger Tiegh. is able to release citric acid up to 300 g/l of nutrient medium under culture conditions (Al-Sheri, Mostafa, 2006), although these amount are generally much lower. The ability to active citric acid excretion was the reason for A. niger usage for industrial acid production (Magnuson, Lasure, 2004; Papagianni, 2007; Pel et al., 2007; Andersen et al., 2011; Show et al., 2015; Almousa et al., 2018; Ozdal, Kurbanoglu, 2019).
On the other hand, organic acids produced by fungi are considered as one of the most important factors in the destruction of various building materials and structures, as well as of cultural heritage (Scheerer, 2009; Warscheid, Braams, 2000; Sterflinger, 2010; Gadd et al., 2014; Boniek et al., 2017).
There is also evidence of the role of fungal organic acids in the processes of pathogenesis in animals and humans. In pulmonary aspergillosis in humans, especially when affected by the fungus A. niger and, less commonly, A. fumigatus Fresen., calcium oxalate crystals form in the lungs. Calcium oxalates are localized in places of the greatest accumulation of mycelium. This phenomenon, called oxalosis, complicates the treatment of aspergillosis and is considered as one of its most dangerous consequences, in especially severe cases leading to the death (Muntz, 1999; Pabuççuolu et al., 2003; Roehrl et al., 2007). Similar phenomena have also been observed in veterinary medicine (Muntz, 1999).
Thus, research on organic acids production by fungi is important for understanding the many ecological and global geochemical processes involving fungi, and has of great practical importance for biotechnology, medicine, and cultural heritage conservation.
In this review we aimed to summarize data about the metabolism and excretion of organic acids by fungi and the functions of organic acids in biogeocenosis.
METABOLISM OF ORGANIC ACIDS
Gluconic acid
Gluconic acid is produced by fungi from D-glucose by the oxidation of its aldehyde group (C1) to a carboxyl group through a dehydrogenation reaction catalyzed by glucose oxidase (unlike glucuronic acid, where C6 is oxidized to a carboxyl group, and glucaric acid, where both C1 and C6 are carboxylic groups). Oxidation of the aldehyde group on the C-1 of beta-D-glucose to a carboxyl group results in the production of glucono-δ-lactone and hydrogen peroxide. Glucono-δ-lactone is further hydrolyzed to gluconic acid either spontaneously or by a lactone-hydrolyzing enzyme (Karaffa et al., 2021; Yadav et al., 2022).
Unlike other acids secreted by fungi, gluconic acid is a product synthesized mainly extracellularly. Depending on the fungal genera, glucose oxidase is either a fully extracellular enzyme or partially cell wall-bound. However, a gene encoding intracellular glucose oxidase was also found in A. niger (Pel et al., 2007). Glucose oxidase is a dimer of two identical subunits containing one FAD molecule each. FAD is restored during glucose oxidation. The subsequent oxidation of FADH2 is accompanied by the formation of hydrogen peroxide, which is decomposed by catalase (Ramachandran et al., 2006).
Gluconic acid, formed by mycelium or added to the medium, is a substrate that is easily absorbed and metabolized by fungi and can be used by them as the sole carbon source (Elzainy et al., 1973; Müller, 1986; Elshafei, 1989). Two gluconate-specific kinases have been identified in A. niger, and it is assumed that gluconic acid catabolism occurs through its phosphorylation to 6-P-gluconate (Pel et al., 2007). Further 6-P-gluconate is included in the pentose phosphate pathway. In addition, gluconic acid can be metabolized in another way, not related to its phosphorylation, where it is dehydrogenated using the enzyme gluconate dehydrogenase, and the 2-keto-3-deoxygluconate formed as a result of this reaction is cleaved by 2-keto-3-deoxy gluconat aldolase into pyruvate and glyceraldehyde (Elzainy et al., 1973; Ramachandran et al., 2006).
The pathways of organic acids biosynthesis in fungi are illustrated on sсheme (Fig. 1).
Fig. 1.
The main pathways of organic acids metabolism in fungi: 1 – glucose oxidase; 2 – gluconolactonase; 3 – gluconate dehydrogenase; 4 – 2-keto-3-deoxy gluconat aldolase; 5 – pyruvate dehydrogenase; 6 – citrate synthase; 7 – aconitase; 8 – cis-aconitate decarboxylase; 9 – isocitrate dehydrogenase; 10 – oxoglutarate dehydrogenase; 11 – succinyl-CoA synthase; 12 – succinate dehydrogenase; 13 – fumarate hydratase; 14 – malate dehydrogenase; 15 – isocitrate lyase; 16 – malate synthase; 17 – lactate de-hydrogenase; 18 – pyruvate carboxylase; 19 – oxaloacetate acetylhydrolase; 20 – glyoxylate dehydrogenase.
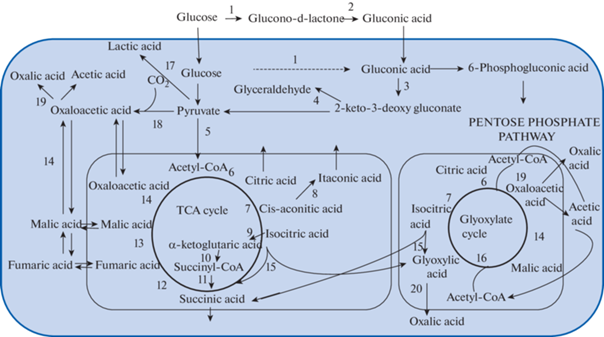
Citric, succinic, fumaric and malic acids
Oxidation of glucose via the glycolytic pathway leads to the formation of pyruvate. With sufficient oxygen, pyruvate is decarboxylated to acetyl-CoA by the mitochondrial pyruvate dehydrogenase complex. Acetyl-CoA then enters the TCA cycle and reacts with oxaloacetate to form citric acid (Peksel et al., 2002). The enzyme citrate synthase, which catalyzes this reaction, is inhibited by Mg2+ and ATP, but not by citric acid (Papagianni, 2007).
Another pathway of pyruvic acid metabolism in fungal cell is also possible. In the 1960s, the fixation of CO2 in fungi by pyruvate carboxylase with the formation of oxaloacetic acid was proposed (Woronick, Johnson, 1960; Bloom, Johnson, 1962). Later one mitochondrial and one cytoplasmic pyruvate carboxylase were found in A. niger (Pel et al., 2007). The resulting oxaloacetic acid, in the case of its synthesis in the cytoplasm, can be transported to mitochondria (through the oxaloacetate translocator in exchange for malate) and included in the TCA cycle. In addition, oxaloacetate can be converted to malic acid by cytoplasmic or mitochondrial malate dehydrogenase (Ma et al., 1981; Pel et al., 2007).
The citric acid formed during the citrate synthase reaction undergoes an isomerization reaction to isocitrate under the aconitase. The cis- aconitic acid formed during this reaction can be decarboxylated by cis-aconitate decarboxylase to form itaconic acid (Bonnarme et al., 1995; Willke, Vorlop, 2001; Magnuson, Lasure, 2004; Pel et al., 2007). Isocitric acid is decarboxylated to α-ketoglutarate by NAD-dependent isocitrate dehydrogenase and undergoes oxidative decarboxylation by oxoglutarate dehydrogenase to form succinyl-CoA. The conversion of succinyl-CoA to succinic acid is catalyzed by the enzyme succinyl-CoA synthase and is accompanied by the formation of ATP. In addition to the oxidation of α-ketoglutarate, the formation of succinic acid is possible by reduction of fumaric acid by succinate dehydrogenase and from isocitrate during the glyoxylate pathway (Munir et al., 2001; Magnuson, Lasure, 2004).
The oxidation of succinic acid to fumaric acid is carried out with the participation of succinate dehydrogenase. A cytoplasmic pathway for the synthesis of fumaric acid from malic acid is also possible due to fumarate hydratase (fumarase) in the cytoplasm. Moreover, in the case of intensive production of fumaric acid by the organism, its activity is high (Carol et al., 2008).
The malic acid, as well as fumaric acid, can be form in mitochondria or in cytoplasm. In the TCA cycle, fumaric acid, under fumarase enzyme, adds water that leads to malic acid formation. Further, malic acid is oxidized by the enzyme malate dehydrogenase to oxaloacetic acid with the formation of NADH (Peksel et al., 2002). Fungi are thought to have three cytoplasmic and one mitochondrial malate dehydrogenases (Pel et al., 2007). Cytoplasmic malate dehydrogenase generates malic acid from oxaloacetic acid synthesized as a result of CO2 fixation by cytoplasmic pyruvate carboxylase (Ma et al., 1981; Magnuson, Lasure, 2004). Further, malate formed in the cytoplasm can be transported to mitochondria, oxidized to oxaloacetate, and condensed with acetyl-CoA to form citric acid (Papagianni, 2007).
Isocitrate lyase catalyzes the reversible cleavage of isocitrate into succinate and glyoxylate and supplies glyoxylate and succinate to the glyoxylate and tricarboxylic acid cycles, respectively. Further, glyoxylate is condensed with acetyl-CoA by malate synthase to form malate, a precursor of oxaloacetate (Munir et al., 2005).
It is assumed that the excretion of di- and tricarboxylic acids occurs with the participation of transport proteins (Gallmetzer et al., 1998; Gallmetzer, Burgstaller, 2002). Odoni et al. (2019) were able to identify a gene encoding the transport protein of citrate transporter in gene encoding the transport protein of citrate transporter in A. niger, but no citrate exporters have yet been identified. It is known the transport systems of malic and succinic acids in most fungi are inhibited by glucose (Jennings, 2007).
With a lack of carbohydrates in the nutrient medium, gluconic, malic, citric, succinic and fumaric acids are consumed by mycelium (Côrte-Real, Leao, 1990; Ghorbani et al., 2007; Sazanova et al., 2016). In this case, the formation of oxalic acid is sometimes observed (Gadd, 1999; Sazanova et al., 2016).
Lactic acid
In case of a lack of oxygen in the cytosol, lactic acid is biosynthesized from pyruvic acid using the enzyme lactate dehydrogenase (Magnuson, Lasure, 2004; Zhang et al., 2007). In Rhizopus oryzae Went et Prins. Geerl., three isoforms of lactate dehydrogenase have been identified: two NAD-dependent and one NAD-independent (Yu, Hang, 1991; Skory, 2000). Molecular studies with gene-specific primers have shown that the two NAD-dependent lactate dehydrogenase genes are differentially expressed. The ldn A gene is expressed in the presence of glucose, xylose, and trehalose. The ldn B gene is expressed only in the presence of ethanol, lactate, and non-fermentable carbon sources. It is assumed that ldn A encodes lactate dehydrogenase, which carries out the reductive reaction pyruvate → lactate, while ldn B encodes lactate dehydrogenase, which catalyzes the oxidative reaction lactate → pyruvate (Magnuson, Lasure, 2004).
Oxalic acid
The mechanism for the formation of oxalic acid from oxaloacetate in Aspergillus niger was first proposed in 1956 by Hayashi et al. (1956). They associated the formation of this acid with the activity of the enzyme oxaloacetate acetylhydrolase (oxaloacetase) (Hayaishi et al., 1956). Since inhibition of TCA cycle in mitochondria did not cause a decrease in the formation of oxalic acid, an assumption was made about the cytoplasmic localization of oxaloacetase. This was later confirmed by fractionation of A. niger mycelial cells (Kubicek et al., 1988).
Hydrolysis of oxaloacetic acid by the cytoplasmic oxaloacetate acetylhydrolase was subsequently recognized as the main pathway for the synthesis of oxalic acid by fungi (Munir et al., 2001; Magnuson, Lasure, 2004; Pel et al., 2007). In addition to A. niger, oxaloacetase activity was found in Sclerotinia sclerotiorum (Lib.) de Bary, Cryphonectria parasitica (Murrill) M.E. Barr, Tyromyces palustris (Berk. et M.A. Curtis) Murrill, Trametes versicolor (L.) Lloyd, and Phanerochaete chrysosporium Burds. (Dutton, Evans, 1996). Subsequent studies showed that oxaloacetase-deficient A. niger strains did not produce oxalic acid (Ruijter et al., 1999). Experiments by Pedersen et al. (2000) and Han et al. (2007) demonstrated that disruption of the oah gene encoding oxaloacetase in A. niger and Botrytis cinerea Pers. leads to the formation of oxalic acid-incapable mutants. As a result, it was suggested that the presence of this gene in fungi is necessary for biosynthesis of oxalate (Pedersen et al., 2000; Han et al., 2007). This oxalic acid biosynthetic pathway does not produce ATP (pyruvate carboxylase requires 1 ATP to form oxaloacetate). The activity of oxaloacetase requires neutralization of the resulting acids and the presence of carbonate in the medium (Kubicek et al., 1988; Gadd, 1999; Pedersen et al., 2000), as well as Mn2+ (Hayaishi et al., 1956; Lenz et al., 1976; Gadd, 1999; Ruijter et al., 1999). Oxaloacetase activity is inhibited by oxalic (Ruijter et al., 1999) and malic acids (Han et al., 2007). Acetate is not an oxaloacetase inhibitor (Ruijter et al., 1999).
As regards the formation of the precursor of oxalic acid, oxaloacetate, its formation in the cytoplasm from pyruvic acid was shown in A. niger, to occur due to the enzyme pyruvate carboxylase (Kubicek et al., 1988). Later it was shown that in wood-destroying fungi, the main part of oxaloacetate is formed by the oxidation of malic acid by malate dehydrogenase (Munir et al., 2001). The acetic acid formed during the hydrolysis of oxaloacetate can be included in the formation of acetyl-CoA and then enter the glyoxylate cycle.
An alternative pathway for the biosynthesis of oxalic acid is its formation from glyoxylic acid with the participation of the enzyme glyoxylate dehydrogenase (Pel et al., 2007; Munir et al., 2001, 2005), although oxaloacetase, apparently, still has a much more contribution (Dutton, Evants, 1996; Munir et al., 2001).
The biochemical role of oxalic acid biosynthesis primarily consists in the oxidation of acetyl-CoA with the formation of oxalate, which accumulates in the culture liquid as the final product. The oxidation of malate to oxaloacetate, the precursor of oxalate, is accompanied by the formation of NADH. The formation of oxalate is thus an energetically favorable process (Munir et al., 2001).
The transport of oxalic acid through the plasmalemma has been studied in detail in Fomitopsis palustris (Berk. et M.A. Curtis) Gilb. et Ryvarden. The results of these studies have shown that active oxalate transport requires Mg and ATP. Vanadate, an inhibitor of P-type ATPases and ABC transporters, significantly reduced the transport of oxalate across the membrane (by 68%). The addition of valinomycin or NH4Cl, which prevent the formation of ∆ψ and ∆pH, inhibited oxalate transport by 86 and 90%, respectively. Inhibitors of ABC transporters, glibenclamide and cyclosporine, did not affect oxalate transport. Thus, it was concluded that secondary oxalate transport occurs in F. palustris, which requires the creation of ∆ψ and ∆pH, while ABC transporters do not perform the leading function in oxalic acid transport (Watanabe et al., 2010).
Unlike most other acids produced by fungi, oxalic acid is not consumed by the mycelium as a nutrient substrate (Espejo, Agsin, 1991). However, fungi have enzyme systems capable of degrading oxalate. The first system includes the oxidation of oxalate by oxalate oxidase with the formation of two CO2 molecules and one H2O2 molecule. This system has been well studied in plants, while in fungi it has been discovered relatively recently and has been shown only in a few species (Dutton, Evants, 1996; Makela et al., 2009; Grąz et al., 2016).
The second system, more common in fungi, involves the decarboxylation of oxalic acid by oxalate decarboxylase to form formic acid and CO2 (Dutton, Evants, 1996; Pel et al., 2007; Makela et al., 2009; Hastrup et al., 2012). It is believed that oxalate decarboxylase is localized mainly inside the mycelium, near the plasma membrane and intracellular vesicles. In the culture liquid of fungi, the cell wall, and in the extracellular polysaccharide layer, its activity is very low (Dutton et al., 1994; Kathiara et al., 2000; Makela et al., 2009). According to other data, in the wood-decay fungus Postia placenta (Fr.) M.J. Larsen et Lombard, the greatest amount of oxalate decarboxylase was observed precisely on the surface of the hyphae of the fungus, while the activity of the enzyme was lower in the extract from the mycelium and in the cultural liquid (Micales, 1997).
Oxalate decarboxylase is a Mn2+ containing enzyme (Makela et al., 2009). The pH optimum for this enzyme is 3.6 and the temperature optimum is 35°C (Kathiara et al., 2000). Under culture conditions, the formation of oxalic acid occurs during the stationary phase, when the pH of the medium is predominantly in the alkaline region, the enzyme activity is suppressed under these conditions, and oxalate decarboxylation occurs weakly. A similar phenomenon is also observed when calcium carbonate is added to the medium (Dutton, Evants, 1996).
It is assumed that the main role of fungal oxalate decarboxylase is to prevent the accumulation of too large amounts of oxalic acid (Micales, 1997; Makela et al., 2009). Oxalate decarboxylase released into the medium degrades extracellular oxalic acid to maintain a certain pH level and oxalate anions outside the cell (Dutton et al., 1994; Micales, 1997; Makela et al., 2009; Schilling, Jellison, 2005).
Oxalate decarboxylase activity is well known for white rot fungi (Makela et al., 2009). Brown rot fungi usually accumulate oxalate in the culture medium and it was assumed that the higher accumulation of oxalic acid observed in brown-rot fungi compared to white-rot fungi is explained by the inability of the former to undertake active regulation of this organic acid (Akamatsu et al., 1992). However, there is evidence that the brown-rot fungi, and in particular Gloeophyllum trabeum (Pers.) Murrill, are capable of regulating oxalic acid concentration during wood decay by decarboxylation (Hastrup et al., 2012).
Schilling and Jellison (2005) demonstrated that brown rot fungi can regulate wood oxalate and pH during decay, but this regulation may be dependent on the environment of decay. The authors could not fully explain the reason for the decrease in the content of oxalates in wood during decay, but the role of the brown rot fungi Meruliporia incrassata (Berk. et M.A. Curtis) Murrill and Fomitopsis pinicola (Sw.) P. Karst. in this process is evident. There is an assumption that the formation of calcium oxalate crystals by brown-rot fungi may neutralize the oxalic acid at the hyphal surface and substitute the need for oxalate-catabolizing enzymes such as oxalate decarboxylase (Micales, 1995).
REGULATION OF BIOSYNTHESIS AND PRODUCTION OF ORGANIC ACIDS BY FUNGI
The intensity of excretion and the quantitative ratio of the organic acids produced by fungi varies significantly depending on growth conditions.
Carbone source
It is believed that the best carbon sources for the production of acids by fungi are mono- and disaccharides, primarily glucose and sucrose (Hossain et al., 1984; Xu et al., 1989; Singh et al., 2001; Papagianni, 2007). The productivity of the formation of oxalic acid by A. niger does not significantly change when glucose, fructose, sucrose, gluconate, xylose, acetate, and glycerol are used as a carbon source (Ruijter et al., 1999). It is known that fungi can synthesize organic acids in significant amounts using other substances. Some strains of A. niger produce oxalic and citric acids at concentrations up to 54 g/l and 36 g/l, respectively, using petroleum as a carbon source (Rymowicz, Lenart, 2002).
According Dörsam et al. (2017), Aspergillus oryzae (Ahlb.) Cohn is able to convert into organic acids a number of sugars including levoglucosan, glucose, galactose, mannose, arabinose, xylose, ribose, cellobiose, fructose, and maltose to malate, albeit in varying yields.
Gluconic acid is produced in greatest quantities using glucose (Singh et al., 2001), although there is evidence that gluconic acid is often formed by fungi using other carbon sources (Ramachandran et al., 2006).
In most cases, the formation of acids by fungi increases with an increase in the concentration of carbohydrates in the medium (Braams, 1992; Gallmetzer, Burgstaller, 2002; Rosling et al., 2004; Magnuson, Lasure, 2004; Al-Shehri, Mostafa, 2006). According to some data, at a sugar concentration of less than 2.5%, the formation of citric acid does not occur (Xu et al., 1989). Our previous study shown that oxalic acid was produced by fungi grown on media with various concentrations of sugars, sugar alcohols, and organic acids. Malic, citric, fumaric, and succinic acids were identified only at elevated carbohydrate concentrations (more than 3%), mostly in liquid cultures. That an increasing concentration of carbohydrates in the medium contributed to the release of acids, which are formed mainly in the TCA cycle and, unlike oxalic acid, are not metabolic end products, but intermediates that can be included in further physiological reactions (Sazanova et al., 2014, 2016). One of the possible mechanisms contributing to the release of these organic acids from the cell in fungi, may be “catabolic inactivation”, i.e. inhibition by sugars in a high concentration of the expression of genes of some respiratory enzymes (Flavell, 1970; Blank, Sauer, 2004; Portnoy, 2011).
In a study by Brunner et al. (2014) it was shown that carbohydrate sources, which contain not only C but also nutrient elements, e.g., algal substrates or pollen material, can trigger better the exudation of organic acids compared to pure and simple carbohydrates such as glucose. Thus, the occurrence of complex carbohydrate sources in nutrient-deficient deglaciated soils may positively influence the exudation of organic acids of fungi.
Nitrogen source
Too low or high concentrations of nitrogen lead to a violation of the growth and development of fungi, as well as reducing acid production. The maximum amount of citric acid in A. niger was obtained at a concentration of about 0.2% NH4NO3 in a medium. Nitrogen deficiency, on the other hand, may promote the oxidation of glucose to gluconic acid by some fungi (Ali et al., 2002a).
In plants, more intensive formation of organic acids (succinate, malate, citrate, fumarate) occurs under conditions of assimilation of predominantly nitrate nitrogen, rather than ammonium nitrogen (Lambers et al., 2008). In fungi, as a rule, a similar dependence is observed. Using the mycorrhizal fungus Paxillus involutus (Batsch) Fr. as an example, it was shown that when using nitrate as a source of nitrogen, a greater release of oxalic acid is observed than when using ammonium (Dutton, Evants, 1996). Although in other studies conducted on A. niger, the use of different nitrogen sources (NaNO3 and NH4Cl) and changing their concentrations from 6 to 50 mmol had almost no effect on the amount of oxalic acid secreted by mycelium (Ruijter et al., 1999).
In addition to the direct influence of nitrogen sources on the growth and formation of acids by fungi, the ratio of carbon and nitrogen in the environment is also important. A high C/N ratio is favorable for the excretion of acids – products of the TCA cycle (Gallmetzer, Burgstaller, 2002; Magnuson, Lasure, 2004; Carol, 2008; Sazanova et al., 2016).
Metal ions
In addition to an excessive level of carbon source, an optimal source of nitrogen, sufficient aeration, the production of organic acids is also dependent on certain trace elements that synergistically affect their production. Their common role in fungal physiology is to provide redox and catalytic activity for a variety of important biochemical reactions as cofactors for enzymes that directly or indirectly affect the biosynthesis of organic acids. Particularly emphasize the importance of five metals Zn, Mn, Mg, Fe, и Cu (Ali et al., 2002b; Papagianni, 2007; Karaffa et al., 2021). Thus, manipulating the supply of cofactors by carefully adjusting the concentration of these metals can fundamentally change the distribution of fluxes in the cell, causing metabolic overload.
The lack of Fe ions, a cofactor of aconitase, increases the formation of citric acid by blocking the subsequent reaction of its transformation in the TCA cycle (Braams, 1992; Carlile et al., 2001). An increase in the concentration of Cu in the medium has a stimulating effect on the synthesis of oxalic, citric and some other acids by fungi (Ramsay et al., 1999; Gibson, Mitсhell, 2004; Fomina et al., 2005; Munir et al., 2005; Betlej, Grąz, 2006). Zn causes an increase in the synthesis of citric acid, and also promotes the synthesis of malic acid (Fomina et al., 2005). Mg has a stimulating effect on the synthesis of oxalic acid, since it is necessary for the functioning of oxaloacetase (Hayaishi et al., 1956; Lenz et al., 1976). In addition, Mn activates isocitrate dehydrogenase (Williams, Pittman, 2010).
The action of metals may vary for different fungi. The addition of Mg to the medium at concentrations of 1 × 10–6–4 × 10–6 leads to an increase in the production of citric acid in some strains and a decrease in its production in others (Ali et al., 2002b). In addition, the influence of elements can manifest itself differently even at the species level, depending on the growth conditions. For example, on nitrate media, zinc stimulates the formation of oxalic acid in Aspergillus niger and Penicillium citrinum Thom, while on ammonium media, it practically does not affect the production of organic acids (Sazanova et al., 2015).
An increase in the concentration of some potentially toxic metals (Cd, Ni, Pb) in the medium stimulates the synthesis of oxalic, citric, malic, and some other acids by fungi (Dutton, Evans, 1996; Ramsay et al., 1999; Gibson, Mitсhell, 2004; Fomina et al., 2005), which seems to be a response to stress.
Acidity
The ratio of organic acids secreted by fungi largely depends on the acidity of the medium. Low acidity promotes the accumulation mainly of oxalic (Ruijter et al., 1999; Carlile et al., 2001; Ali et al., 2002a; Al-Shehri, Mostafa, 2006) and gluconic acids (Braams, 1992). Optimal for the biosynthesis of gluconic acid is pH 6.5. At a nutrient solution pH of less than 3.5, the work of the glucose oxidase enzyme is completely inactivated (Anastassiadis, Rehm, 2006). The acidity of the extracellular solution is one of the main regulators of oxaloacetase activity. The activity of this enzyme is suppressed by low pH (Kubicek et al., 1988; Ruijter et al., 1999). It is believed that high acidity induces the formation of citric acid (Carlile et al., 2001; Zhang, Röhr, 2002a, 2002b). It is possible that the formation of citrate is enhanced at high acidity of the medium due to a decrease in carbon consumption for the formation of oxalic and gluconic acids (Ruijter et al., 1999). A. niger mutants lacking glucose oxidase (gox C) and oxaloacetase (prt F) produced citric acid in large amounts and at neutral pH. The most intensive formation of lactic and fumaric acids of Rhizopus sp. observed at pH 6.0–6.5 and decreases with increasing acidity (Zhang et al., 2007).
Temperature
The temperature optimum for the formation of most acids is 25–30°C (Ali et al., 2002a; Haq et al., 2002; Al-Sheri, Mostafa, 2006; Anastassiadis, Rehm, 2006; Zhang et al., 2007). At extremely high temperatures at 40°C, the biosynthesis of oxalic acid is activated, while the formation of citric and some other acids is suppressed (Haq et al., 2002).
UV irradiation
In most cases the metabolic response of fungi to stressful exposure under UV light is to increase the production of citric acid by fungi (Vasanthabharathi et al., 2013), although in some strains exposed to long-term exposure to UV radiation, the production of citric acid is reduced (Tembhurkar et al., 2012). In cultures of micromycetes Aspergillus niger, Rhodotorula colostri (T. Castelli) Lodder, and Geomyces pannorum (Link) Sigler et J.W. Carmich. irradiated by UV light, the intensity of oxalic acid production increased. Unlike Aspergillus niger and Rhodotorula colostri, Geomyces pannorum also increased the production of succinic, fumaric, and malic acids under UV influence (Sazanova et al., 2014).
UV along with chemical mutagens (for example, sodium azide, ethidium bromide, aziridine, N-nitroso-N-methylurea, ethyl methanesulfonate), is used to select strains that are hyperproducers of organic acids (Musilkova et al., 1983; Tembhurkar et al., 2012). In mutant strains obtained by prolonged exposure to UV light, gluconic acid biosynthesis is stimulated due to the activation of the glucose oxidase enzyme (Prabu et al., 2012). Strains of Apergillus niger – hyperproducers of citric acid have mutations in the genes associated with plasma membrane ATPase, the TCA cycle, and in the components of the electron transport chain (Andersen et al., 2011). An increase in the biosynthesis of acids-intermediates of the TCA cycle was also observed in plants exposed to UV irradiation (Kim et al., 2012).
ECOLOGICAL ROLE OF FUNGAL ACID PRODUCTION
Role of organic acids in wood decay
Wood-decaying fungi, especially brown rot, produce oxalic acid in large quantities (Espejo, Agosin, 1991; Dutton et al., 1993; Munir et al., 2005; Makela et al., 2009; Jarosz-Wilkołazka, Grąz, 2006).
In brown rot fungi, the formation of oxalic acid is most likely associated with its function of reducing Fe (III) to Fe (II) and its subsequent participation in the depolymerization of wood cellulose due to participation in Fenton reactions (Espejo, Agosin, 1991). Oxalic acid produced by brown rot fungi plays an important role in the Fenton reaction due to its ability to bind and dissolve iron from Fe oxyhydroxide complexes in the wood lumen region. Conditions of pH can strongly influence the dissolution of iron oxides (Arantes et al., 2012).
In white rot fungi, oxalate and malonate, in lesser degree promote the work of manganese peroxidases that are part of the ligninolytic system (Dutton et al., 1993; Kuan, Tien, 1993; Dutton, Evants, 1996; Çaliskan, 2000; Munir et al., 2005). During the catalytic cycle, the active center is oxidized by H2O2. Reduction to the resting enzyme is achieved by two consecutive one-electron transfers, as a result of which Mn2+ is oxidized to Mn3+, respectively. This is promoted by action of organic acids during chelation of the highly reactive Mn3+ state (Schlosser, Höfer, 2002).
Organic acid – a virulence factor for pathogenic fungi
The secretion of organic acids is important during the fungal invasion and pathogenesis process, since the environmental pH effectively regulates the growth and development of fungi, promotes the penetration of hyphae into plant tissues, and also increases their virulence and pathogenicity (Prusky et al., 2008). Organic acid molecules secreted by acidic fungi are multifunctional, including activating virulence factors and enhancing the pathogenicity of certain fungi (Jiao et al., 2022).
Penicillium spp. mainly secrete gluconic and citric acids (Prusky et al., 2004; Hadas et al., 2007). For phytopathogenic fungi Sclerotium rolfsii Sacc., Sclerotinia sclerotiorum, Rhizoctonia solani J.G. Kühn, and Botrytis cinerea, oxalic acid is one of the key pathogenic factors (Cessna et al., 2000; Rollins, Dickman, 2001; Manteru et al., 2003; Prusky, Lichter, 2008). There is evidence that the intensity of organic acid biosynthesis correlates with the pathogenicity and virulence of phytopathogenic fungi (Kunz et al., 2006; Barad et al., 2012; Liang et al., 2015). For example, the transcription factor (pacC) mutant of Aspergillus carbonarius (Bainier) Thom is unable to effectively acidify the nutrient medium or infect its host as a result of reduced production of gluconic and citric acids (Barda et al., 2020).
The main role of organic acids in the process of pathogenesis is associated with acidification of the environment. Generally, an acidic pH is optimal for extracellular cell wall degrading enzymes such as cellulase, hemicellulase and pectinase produced by phytopathogenic fungi (Prusky et al., 2004; Hadas et al., 2007). In addition, the toxic effect of oxalic acid itself leads to tissue damage and plant cell death (Magro et al., 1984; Verhoeff et al., 1988; Pinna, 1993; Dutton, Evants, 1996). Oxalic acid inhibits polyphenol oxidase activity in plants and also induces the formation of reactive oxygen species (Xiao-ting et al., 2009) and intensifies programmed cell death in plants (Williams et al., 2011). In addition, oxalic acid inhibits early defense responses in the host plant (Cessna et al., 2000; Williams et al., 2011).
Oxalic acid binding of Ca2+ ions in the plant cell wall can also facilitate the penetration of fungi into plant tissues (Pinna, 1993; Dutton, Evants, 1996; Hadas et al., 2007). Oxalic acid chelates Ca2+ to form insoluble calcium oxalate crystals that erode cell walls causing host plant wilt (Cessna et al., 2000; Prusky, Lichter, 2008).
Chelation of Ca2+ by citric acid is also to impair host cell wall function and lead to cell death. The accumulation of citric acid reduces Ca2+ activity between plant cells, changing the mineral balance and affecting the stability of cell membranes and cell wall pectin polymers (Jiao et al., 2022).
The role of organic acids in the formation of mycorrhiza
The production of organic acids is also a common feature of many ectomycorrhizal fungi (Malajczuk, Cromack, 1982; Dutton, Evants, 1996; Rosling et al., 2004; Kanwal, 2006). It is believed that the oxalic acid secreted by them is essential in the formation of mycorrhiza, contributing to the trophic connection between the root and hyphae of fungi (Malajczuk, Cromack, 1982; Dutton, Evants, 1996). In some cases, the predominant acids secreted by ectomycorrhizal fungi are citrate and malate (Nowotny et al., 1998; Blaudez et al., 2000). It is assumed that the release of oxalic acid by the mycorrhizal fungus Paxillus involutus has a toxic effect on pathogenic fungi in the rhizosphere, thus contributing to the protection of the roots of Pinus sp. It has also been noted that pine root exudates stimulate the production of oxalic acid by P. involutus (Allen et al., 1992).
The role of organic acids in geochemical cycles
Organic acids exudation by fungi play a major role in mineral weathering contributing to the geochemical carbon cycle. The acidification ability largely determines the geochemical role of fungi and their importance in rock weathering and primary soil formation processes (Ferris et al., 1994; Vaughan et al., 2002). Organic acids play an important role in changing the solubility of compounds (Nowotny et al., 1998; Wallander, Wickman, 1999; Blaudez et al., 2000; Rosling et al., 2004; Kanwal, 2006). Acidification of the environment promotes the extraction of elements such as P, K, Al, Fe from minerals, and the transfer of some of them into forms more accessible to organisms (Hoffland et al., 1992; Jones, Darrah, 1995; Ohwaki et al., 1997; Ghorbani et al., 2007; Posso et al., 2017). It is well known that acids secreted by plant roots can have a similar effect (López-Bucio, 2000).
The secretion of oxalic acid is believed to play a major role in the dissolution of apatite, the release of phosphorus for uptake by ectomycorrhizal fungi, and the immobilization of the released calcium into calcium oxalate (Smits et al., 2012).
The primary mechanisms of organic acid interaction with metals include acidolysis, complexolysis, bioaccumulation, and chelate formation (Blaudez et al., 2001; Rosling et al., 2004; Kanwal, 2006; Dusengemungu et al., 2021).
An intense release of oxalic acid is often observed in fungi living on substrates with a high Ca2+ content (Dutton, Evans, 1996; Cezar, 1998; Adamo, Violante, 2000; Kolo, Claeys, 2005). Fungi, as well as plants growing in alkaline soils rich in calcium, can use organic acids to bind excess calcium. Calcium chelation is critical for survival in soils with high calcium concentrations that disrupt several cell metabolic processes such as calcium-dependent signaling, phosphorus metabolism, and cytoskeletal organization (Dutton, Evans, 1996; Adamo, Violante, 2000; López-Bucio, 2000).
Oxalic acid production by fungi is a powerful factor in modern mineral formation. Abundant formation of calcium oxalate crystals can be observed in fungal cultures (Monte, 2003; Gadd et al., 2014; Sturm et al., 2015). Oxalic acid forms insoluble salts with other divalent and trivalent metals. Zn, Cu, Fe and Pb oxalates were obtained in experimental controlled conditions under the influence of Aspergillus niger (Vlasov et al., 2020) as well as some other species (Fomina et al., 2005). However, most often and in the largest quantity, fungi form calcium oxalate. In the case of ectomycorrhizal fungi, it has been shown, the main chemical sink for secreted oxalate is the formation of crystaline calcium oxalate, irrespective of the chemistry of the minerals (Schmalenberger et al., 2015).
The role of organic acids in adaptation to heavy metals
Despite the fact that oxalates of potentially toxic heavy metals such as Fe, Zn, Pb, Cu, Cd, Ni and some others are formed by fungi much less frequently than calcium oxalates, among the mechanisms that ensure the resistance of fungi to heavy metals, an important role belongs to the extracellular chelation of metal ions by secreted acids (Gadd, 1993; Dutton, Evans, 1996; Gadd, 1999; Ramsay et al., 1999; Gibson, Mitсhell, 2004; Fomina et al., 2005; Munir et al., 2005). The formation of complexes of organic acids with Al, Fe, Mg, Mn, Zn, Pb, Cu reduces the activity and toxicity of these metals in the soil or on another substrate and promotes the survival of fungi, as well as other organisms in an environment with their high content (Dutton, Evans, 1996; Gadd, 1999; Ramsay et al., 1999; Ruijter et al., 1999; Hoffland et al., 2004; Gibson, Mitсhell, 2004; Fomina et al., 2005; Munir et al., 2005; Kanwal, 2006). The production of oxalic acid, which forms stable complexes and/or insoluble salts with a number of metals, is considered as a highly efficient method for the immobilization of toxic metals (Munir et al., 2005; Jenkins et al., 2012). In association with plants, the production of organic acids by fungi contributes to the resistance of plants to the heavy metals (Ahonen-Jonnarth, 2000).
CONCLUSION
The metabolism and excretion of organic acids by fungi is a very labile process which depends on many factors. Nutrient sources and the mineral composition of the substrate are the key importance. Increased production of organic acids by fungi is often a response to stress including heat, ultraviolet, and heavy metals. In nature, the production of organic acids by fungi favors for their assimilation of various substrates. Organic acids are involved in the formation of mycorrhiza, the processes of plant pathogenesis, and are also one of the key factors in wood decay. By changing the bioavailability of elements and the solubility of minerals, organic acids produced by fungi are very significant for the functioning of ecosystems.
This study was supported by the Russian Science Foundation (Project No. 21-74-00031 “Fungi and bacteria in biogeochemical cycles: trophic and allelopathic interactions, role in metal detoxification”).
Список литературы
Adamo P., Violante P. Weathering of rocks and neogenesis of minerals associated with lichen activity. Applied Clay Science. 2000. V. 16. P. 229–256. https://doi.org/10.1016/S0169-1317(99)00056-3
Ahonen-Jonnarth U., Van Hees P.A.W., Lundstrom U.S. et al. Organic acids produced by mycorrhizal Pinus sylvestris exposed to elevated aluminium and heavy metal concentrations. New Phytol. 2000. V. 146 (3). P. 557–567.
Akamatsu Y., Takahashi M., Shimada M. Cell-free extraction and assay of oxaloacetate from the brown-rot fungus Tyromyces palustris. Mokuzai Gak-kaishi, Japan Wood Research Society. 1992. 38. 495e500.
Ali S., Haq I., Iqbal J. The role of Mn++ ions for high and consistent yield of citric acid in recycling fed-batch bioreactor system and its novelty on kinetic basis. Electronic J. Biotechnol. 2002b. V. 5 (2). https://doi.org/10.4067/S0717-34582002000200005
Ali S., Haq I., Qadeer M.A., Iqbal J. Production of citric acid by Aspergillus niger using cane molasses in a stirred fermentor. Electronic J. Biotechnol. 2002a. V. 5 (3). https://doi.org/10.2225/vol5-issue3-fulltext-3
Allen M. Mycorrhizal functioning: An integrative plant-fungal process. Chapman and Hall, N.Y., 1992.
Almousa A.A., El-Ghany A.M.N., Ashour E.H. Citric acid fermentation by Aspergillus niger. J. Innovations in Pharm. and Biol. Sci. 2018. V. 5 (4). P. 20–37.
Al-Shehri A.M., Mostafa Y.S. Citric acid production from date using syrop immobilized cells of Aspergillus niger. Biotechnology. 2006. V. 5 (4). P. 461–465. https://doi.org/10.3923/biotech.2006.461.465
Anastassiadis S., Rehm H.-J. Continuous gluconic acid production by Aureobasidium pullulans with and without biomass retention. Electronic J. Biotechnol. 2006. V. 9 (5). https://doi.org/10.2225/vol9-issue5-fulltext-18
Andersen M.R., Salazar M.P., Schaap P.J. et al. Comparative genomics of citric-acid-producing Aspergillus niger ATCC 1015 versus enzyme-producing CBS 513.88. Genome Res. 2011. V. 21 (6). P. 885–897. https://doi.org/10.1101/gr.112169.110
Arantes V., Jellison J., Goodell B. Peculiarities of brown-rot fungi and biochemical Fenton reaction with regard to their potential as a model for bioprocessing biomass. Appl. Microbiol. Biotechnol. 2012. V. 94. P. 323–338. https://doi.org/10.1007/s00253-012-3954-y
Barad S., Horowitz S.B., Moscovitz O. et al. A Penicillium expansum glucose oxidase-encoding gene, GOX2, is essential for gluconic acid production and acidification during colonization of deciduous fruit. Molec. Plant-Microbe Interact. 2012. V. 25. P. 779–788. https://doi.org/10.1094/MPMI-01-12-0002
Barda O., Maor U., Sadhasivam S. et al. The pH-responsive transcription factor PacC governs pathogenicity and ochratoxin A biosynthesis in Aspergillus carbonarius. Frontiers in Microbiology. 2020. V. 11. 210. https://doi.org/10.3389/fmicb.2020.00210
Bennet-Clark T.A. The role of organic acids in plant metabolism. State publishing house of biological and medical literature, Leningrad, 1938 (in Russ.)
Betlej I., Grąz M. The identification of organic acid in Tra-metes versicolor culture, growing on a medium with CuHDO complex. Folia Forestalia Polonica. 2006. B. V. 37. P. 3–7.
Blank L.M., Sauer U. TCA cycle activity in Saccharomyces cerevisiae is a function of the environmentally determined specific growth and glucose uptake rates. Microbiology. 2004. V. 150. P. 1085–1093. https://doi.org/10.1099/mic.0.26845-0
Blaudez D., Jacob C., Turnau K. et al. Differential responses of ectomycorrhizal fungi to heavy metals in vitro. Mycol. Res. 2000. V. 104. P. 1366–1371. https://doi.org/10.1017/S0953756200003166
Bloom S.J., Johnson M.J. The pyruvate carboxylase of Aspergillus niger. J. Biol. Chemistry. 1962. V. 237. P. 2718–2720.
Boniek D., MendesIsolda I.C., Abreu C.M. et al. Ecology and identification of environmental fungi and metabolic processes involved in the biodeterioration of Brazilian soapstone historical monuments. Lett. Appl. Microbiol. 2017. V. 65. P. 1–8. https://doi.org/10.1111/lam.12794
Bonnarme P., Gillet B., Sepulchre A.M. et al. Itaconate biosynthesis in Aspergillus terreus. J. Bacteriol. 1995. P. 3573–3578.
Braams J. Ecological studies on the fungal microflora inhabiting historical sandstone monuments: PhD thesis. Oldenburg, 1992.
Brunner I., Goren A., Schlumpf A. Patterns of organic acids exuded by pioneering fungi from a glacier forefield are affected by carbohydrate sources. Env. Res. Lett. 2014. V. 9. https://doi.org/10.1088/1748-9326/9/2/025002
Butkevich V.S. Selected works. Publishing House of the Academy of Sciences, Moscow, 1957 (in Russ.).
Çaliskan M. The metabolism of oxalic acid. Turkish J. Zool. 2000. V. 24. P. 103–106.
Carlile M.J., Watkinson S.C., Gooday G.W. The Fungi. Academic press, San Diego, 2001.
Carol A., Engel R., Straathof A.J. et al. Fumaric acid production by fermentation. Appl. Microbiol. Biotechnol. 2008. V. 78 (3). P. 379–389. https://doi.org/10.1007/s00253-007-1341-x
Cessna S.G., Sears V.E., Dickman M.B. et al. Oxalic acid, a pathogenicity factor for Sclerotinia sclerotiorum, suppresses the oxidative burst of the host plan. The Plant Cell. 2000. 12. P. 2191–2200. https://doi.org/10.1105/tpc.12.11.2191
Cezar T.M. Calcium oxalate: A surface treatment for limestone. J. Conservation and Museum Studies. 1998. V. 4. https://doi.org/10.5334/jcms.4982
Côrte-Real M., Leão C. Transport of malic acid and other dicarboxylic acids in the yeast Hansenula anomala. Appl. Environm. Microbiol. 1990. V. 56 (4). P. 1109–1113.
Dörsam S., Fesseler J., Gorte O. et al. Sustainable carbon sources for microbial organic acid production with filamentous fungi. Biotechnol. Biofuels. 2017. 10 (242). https://doi.org/10.1186/s13068-017-0930-x
Dusengemungu L., Kasali G., Gwanama C. et al. Overview of fungal bioleaching of metals. Environm. Advances. 2021. V. 5. 100083. https://doi.org/10.1016/j.envadv.2021.100083
Dutton M.V., Evans C.S. Oxalate production by fungi: Its role in pathogenicity and ecology in the soil environment. Can. J. Microbiol. 1996. V. 42. P. 881–895. https://doi.org/10.1139/m96-114
Dutton M.V., Evans C.S., Atkey P.T. et al. Oxalate production by Basidiomycetes, including the white-rot species Coriolus versicolor and Phanerochaete chrysosporium. Appl. Microbiol. Biotechnol. 1993. № 39. P. 5–10.
Dutton M.V., Kathiara M., Gallagher I.M. et al. Purification and characterization of oxalate decarboxylase from Coriolus versicolor. FEMS Microbiol Lett. 1994. V. 116. P. 321–326. https://doi.org/10.1016/j.ibiod.2012.05.030
Elshafei A.M. Degradation of some sugars and sugar acids by the nonphosphorylated D-gluconate pathway in Aspergillus ustus. Acta Biotechnologica. 1989. V. 9 (5). P. 485–489. https://doi.org/10.1002/abio.370090523
Elzainty T.A., Hassan M.M., Allam A.M. New pathway for nonphosphorylated degradation of gluconate by Aspergillus niger. J. Bacteriol. 1973. V. 114 (1). P. 457–459. https://doi.org/10.1128/jb.114.1.457-459.1973
Espejo E., Agosin E. Production and degradation of oxalic acid by brown rot fungi. Appl. Environm. Microbiol. 1991. V. 57 (7). P. 1980–1986. https://doi.org/10.1128/aem.57.7.1980-1986.1991
Ferris F.G., Wiese R.G., Fyfe W.S. Precipitation of carbonate minerals by microorganisms: implications for silicate weathering and the global carbon dioxide budget. Geomicrobiology J. 1994. V. 12. P. 1–13. https://doi.org/10.1080/01490459409377966
Flavell R.B. The regulation of synthesis of Krebs cycle enzymes in Neurospora by catabolite and end product repression. Eur. J. Biochem. 1970. V. 13. P. 548–663. https://doi.org/10.1111/j.1432-1033.1970.tb00959.x
Fomina M., Hillier S., Charnock J.M. et al. Role of oxalic acid overexcretion in transformations of toxic metal minerals by Beauveria caledonica. Appl. Environm. Microbiol. 2005. V. 71 (1). P. 371–381. https://doi.org/10.1128/AEM.71.1.371-381.2005
Franzen H., Schmitt F. Die Bilding der Citronensaure aus Ketipinsaure. Ber. D. D. Chem. 1938. Ges. 58. P. 222.
Gadd G.M. Fungal production of citric and oxalic acid: Importance in metal speciation, physiology and biogeochemical processes. Advances in Microbial Physiology. 1999. V. 41. P. 47–91. https://doi.org/10.1016/S0065-2911(08)60165-4
Gadd G.M. Interactions of fungi with toxic metals. New Phytol. 1993. V. 124. P. 25–60.
Gadd G.M., Bahri-Esfahani J., Li Q. et al. Oxalate production by fungi: Significance in geomycology, biodeterioration and bioremediation. Fungal Biology Reviews. 2014. V. 28. P. 36–55. https://doi.org/10.1016/j.fbr.2014.05.001
Gallmetzer M., Brigitte M., Burgstaller W. Net efflux of citrate in Penicillium simplicissimum is mediated by a transport protein. Archives of Microbiology. 1998. V. 169. P. 353–359. https://doi.org/10.1007/s002030050582
Gallmetzer M., Burgstaller W. Efflux of organic acids in Penicillium simplicissimum is an energy-spilling process, adjusting the catabolic carbon flow to the nutrient supply and the activity of catabolic pathways. Microbiology. 2002. V. 148. P. 1143–1149. https://doi.org/10.1099/00221287-148-4-1143
Ghorbani Y., Oliazadeh M., Shahvedi A. et al. Use of some isolated fungi in biological Leaching of Aluminum from low grade bauxite. African Journal of Biotechnology. 2007. V. 6 (11). P. 1284–1288.
Gibson B.R., Mitchell D.T. Nutritional influences on the solubilization of metal phosphate by ericoid mycorrhizal fungi. Mycol. Res. 2004. V. 108 (8). P. 947–954. https://doi.org/10.1017/s095375620400070x
Grąz M., Rachwał K., Zan R. et al. Oxalic acid degradation by a novel fungal oxalate oxidase from Abortiporus biennis. Acta Biochimica Polonica. 2016. Vol. 63 (3). P. 595–600. https://doi.org/10.18388/abp.2016_1282
Hadas Y., Goldberg I., Pines O. et al. Involvement of gluconic acid and glucose oxidase in the pathogenicity of Penicillium expansum in Apples. Phytopatology. 2007. V. 97 (3). P. 384–390. https://doi.org/10.1094/PHYTO-97-3-0384
Hamlet W.M., Plowright C.B. On occurrence of oxalic acid in fungi. Chemistry News. 1877. V. 36 (927). P. 93–94.
Han Y., Joosten H-J., Niu W. et al. Oxaloacetate hydrolase, the C–C bond lyase of oxalate secreting fungi. The journal of biological chemistry. 2007. V. 282 (13). P. 9581–9590. https://doi.org/10.1074/jbc.M608961200
Haq I., Ali S., Qadeer M.A. et al. Citric acid fermentation by mutant strain of Aspergillus niger GCMC-7using molasses based medium. Electronic J. Biotechnol. 2002. V. 5 (2).
Hastrup A.C.S., Green III F., Lebow P.K. et al. Enzymatic oxalic acid regulation correlated with wood degradation in four brown-rot fungi. Int. Biodeteriorat. Biodegradation. 2012. V. 75. P. 109–114. https://doi.org/10.1016/j.ibiod.2012.05.030
Hayaishi O., Shimazo H., Katagiri M. et al. Enzymatic formation of oxalate and acetate from oxaloacetate. J. Amer. Chem. Soc. 1956. V. 78. P. 5126–5127. https://doi.org/10.1021/ja01600a084
Hoffland E., Kuyper T.W., Wallander H. et al. The role of fungi in weathering. Frontiers in Ecology and the Environment. 2004. V. 2 (5). P. 258–264. https://doi.org/10.1890/1540-9295(2004)002[0258:TROFIW]2.0.CO;2
Hoffland E., Van den Boogard R., Nelemans J. et al. Biosynthesis and root exudation of citric and malic acids in phosphate-starved rape plants. New Phytol. 1992. V. 122. P. 675–680.
Hossain M., Brooks J.D., Maddox I.S. The effect of the sugar source on citric acid production by Aspergillus niger. Appl. Microbiol. Biotechnol. 1984. V. 19. P. 393–397.
Jarosz-Wilkołazka A., Grąz M. Organic acids production by white rot Basidiomycetes in the presence of metallic oxides. Can. J. Microbiol. 2006. 52. P. 779–785. https://doi.org/10.1139/W06-032
Jenkins K.M. Evaluating the mechanism of oxalate synthesis of Fibroporia radiculosa isolates adapting to copper-tolerance. PhD thesis. Forest Products in the Department of Forest Products, Mississippi, 2012.
Jennings D.H. The physiology of fungal nutrition. Cambridge University Press, Cambridge, 2007.
Jiao W., Liu X., Li Y. et al. Organic acid, a virulence factor for pathogenic fungi, causing postharvest decay in fruits. Molec. Plant Pathol. 2022. 23 (2). P. 304–312. https://doi.org/10.1111/mpp.13159
Jones D.L., Darrah P.R. Influx and efflux of organic acids across the root-soil interface of Zea mays L. and its implications in rhizosphere C flow. Plant and Soil. 1995. V. 173. P. 103–109.
Kanwal H.K. Organic acid exudation by ectomycorrhizal fungi in response to aluminium. Master’s thesis. Patiala, 2006.
Karaffa L., Fekete E., Kubicek C.P. The role of metal ions in fungal organic acid accumulation. Microorganisms. 2021. V. 9 (6). 1267. https://doi.org/10.3390/microorganisms9061267
Kathiara M., Wood D.A., Evans C.S. Detection and partial characterization of oxalate decarboxylase from Agaricus biosporus. Mycol. Res. 2000. V. 104. P. 345–350. https://doi.org/10.1017/S095375629900129X
Kim S., Yun E.J., Hossain M.A. et al. Global profiling of ultraviolet-induced metabolic disruption in Melissa officinalis by using gas chromatography-mass spectrometry. Analytical and Bioanalytical Chem. 2012. V. 404 (2). P. 553–562. https://doi.org/10.1007/s00216-012-6142-0
Kolo K., Claeys Ph. In vitro formation of Ca-oxalates and the mineral glushinskite by fungal interaction with carbonate substrates and seawater. Biogeosciences Discuss. 2005. V. 2. P. 277–293. https://doi.org/10.5194/bg-2-277-2005
Kuan I.C., Tien M. Stimulation of Mn peroxidase activity: a possible role for oxalate in lignin biodegradation. PNAS USA. 1993. V. 90. P. 1242–1246. https://doi.org/10.1073/pnas.90.4.1242
Kubicek C.P., Schreferl-Kunar G., Wöhrer W. et al. Evidence for a cytoplasmic pathway of oxalate biosynthesis in Aspergillus niger. Appl. Environm. Microbiol. J. 1988. V. 54. P. 633–637.
Kunz C., Vandelle E., Rolland S. et al. Characterization of a new, nonpathogenic mutant of Botrytis cinerea with impaired plant colonization capacity. New Phytol. 2006. V. 170. P. 537–550. https://doi.org/10.1111/j.1469-8137.2006.01682.x
Lambers H., Chapin III F.S., Pons T.L. Plant physiological ecology. 2nd ed. Springer, N.Y., 2008.
Lenz H., Wunderwald P., Eggerer H. Partial purification and some properties of oxaloacetase from Aspergillus niger. Eur. J. Biochem. 1976. V. 65. P. 225–235. https://doi.org/10.1111/j.1432-1033.1976.tb10409.x
Liang X.F., Liberti D., Li M. et al. Oxaloacetate acetylhydrolase gene mutants of Sclerotinia sclerotiorum do not accumulate oxalic acid but do produce limited lesions on host plants. Molec. Plant Pathol. 2015. V. 16. P. 559–571. https://doi.org/10.1111/mpp.12211
López-Bucio J., Nieto-Jacobo M.F., Ramírez-Rodríguez V. et al. Organic acid metabolism in plants: from adaptive physiology to transgenic varieties for cultivation in extreme soils. Plant Science. 2000. V. 160. P. 1–13. https://doi.org/10.1016/s0168-9452(00)00347-2
Ma H., Kubicek C.P., Rohr M. Malate dehydrogenase isoenzymes from Aspergillus niger. FEMS Microbiology Letters. 1981. V. 12. P. 147–151. https://doi.org/10.1111/j.1574-6968.1981.tb07630.x
Magnuson J.K., Lasure L.L. Organic acid production by filamentous fungi. In: Advances in fungal biotechnology for industry, agriculture, and medicine. 2004. P. 307–340. https://doi.org/10.1007/978-1-4419-8859-1_12
Magro P., Marciano P., Lenna P.D. et al. Oxalic acid production and its role in pathogenesis of Sclerotinia sclerotiorum. FEMS Microbiology Letters. 1984. V. 24. P. 9–12. https://doi.org/10.1111/j.1574-6968.1984.tb01234.x
Makela M.R., Hilden K., Hatakka A. et al. Oxalate de-carboxylase of the white-rot fungus Dichomitus squalens demonstrates a novel enzyme primary structure and non-induced expression on wood and in liquid cultures. Microbiology. 2009. V. 155. P. 2726–2738. https://doi.org/10.1099/mic.0.028860-0
Malajczuk N., Cromack K. Accumulation of calcium oxalate in the mantle of ectomycorrhizal roots of Pinus radicata and Eucalyptus marginata. New Phytol. 1982. V. 92. P. 527–531.
Manteru S., Abouna S., Lambert B. et al. Differential regulation by ambient pH of putative virulence factors secretion by the phytopathogenic fungus Botrytis cinerea. FEMS Microbiol. Ecol. 2003. V. 43. P. 359–366. https://doi.org/10.1111/j.1574-6941.2003.tb01076.x
Max B., Salgado J.M., Rodríguez N. et al. Biotechnological production of citric acid. Brazilian J. Microbiol. 2010. V. 41 (4). P. 862–875. https://doi.org/10.1590/S1517-83822010000400005
Micales J. A. Localization and induction of oxalate decarboxylase in the brown-rot wood decay fungus Postia placenta. Int. Biodeterior. Biodegradation. 1997. V. 39. P. 125–132. https://doi.org/10.1016/S0964-8305(97)00009-7
Micales J.A. Oxalate decarboxylase in the brown-rot wood decay fungus Postia placenta. Material und Organismen. 1995. V. 29. P. 177–186.
Monte M. Oxalate film formation on marble specimens caused by fungus. J. Cultural Heritage. 2003. V. 4. P. 255–258. https://doi.org/10.1016/S1296-2074(03)00051-7
Müller H.M. Utilization of gluconate by Aspergillus niger. II. Enzymes of degradation pathways and main end products. Zentralblatt für Mikrobiologie. 1986. V. 141 (6). P. 461–469.
Munir E., Hattori T., Shimada M. Role for oxalate acid biosynthesis in growth of copper tolerant wood-rotting and pathogenic fungi under environmental stress. The 55th meeting of the Japan wood research society. 2005. P. 1–7.
Munir E., Yoon J.J., Tokimatsu T. et al. New role for glyoxylate cycle enzymes in wood-rotting basidiomycetes in relation to biosynthesis of oxalic acid. J. Wood Science. 2001. V. 47. P. 368–373.
Muntz F.H.A. Oxalate-producing pulmonary aspergillosis in an Alpaca. Veterinary Pathol. 1999. V. 36. P. 631–632. https://doi.org/10.1354/vp.36-6-631
Musilkova M., Ujcova E., Seichert L. et al. Effect of changed cultivation conditions on themorphology of Aspergillus niger and citric acid biosynthesis in laboratory cultivation. Folia Microbiol. 1983. V. 27. P. 382–332. https://doi.org/10.1007/BF02883134
Nowotny J., Schwanz J., Rothe G.M. Influence of soil acidification and liming on selected enzymes of the carbohydrate metabolism and the contents of two major organic acids of mycorrhizal roots of Norway spruce (Picea abies). Plant and Soil. 1998. V. 199. P. 41–51.
Odoni D.I., Laothanachareon T., Vazquez-Vilar M. et al. Aspergillus niger citrate exporter revealed by comparison of two alternative citrate producing conditions. FEMS Microbiology Letters. 2019. 366 (7). https://doi.org/10.1101/259051
Ohwaki Y., Sugahara K. Active extrusion of protons and exudation of carboxylic acids in response to iron deficiency by roots of chickpea (Cicer arietinum L.). Plant Soil. 1997. V. 189. P. 49–55.
Ozdal M., Kurbanoglu E.B. Citric Acid Production by Aspergillus niger from agro-industrial by-products: molasses and chicken feather peptone. Waste and Biomass Valorization. 2019. 10. P. 631–640.
Pabuççuolu H.U., Özkara E., Ceylan E. et al. Oxalosis around a pulmonary fungus ball (aspergilloma). Turkish Respiratory J. 2003. V. 4 (1). P. 21–24.
Papagianni M. Advances in citric acid fermentation by Aspergillus niger: biochemical aspects, membrane transport and modeling. Biotechnology Advances. 2007. V. 25 (3). P. 244–263. https://doi.org/10.1016/j.biotechadv.2007.01.002
Pedersen H., Christensen B., Hjort C. et al. Cloning and characterization of oah, the gene encoding oxaloacetate hydrolase in Aspergillus niger. Molecular Genetics and Genomics. 2000. V. 263. P. 281–286. https://doi.org/10.1007/s004380051169
Peksel A., Torres N.V., Liu J. et al. 13C-NMR Analysis of glucose metabolism during citric acid production by Aspergillus niger. Appl. Microbiol. Biotechnol. 2002. V. 58. P. 157–163. https://doi.org/10.1007/s00253-001-0839-x
Pel H.J., de Winde J.H., Archer D.B. et al. Genome sequen-cing and analysis of the versatile cell factory Aspergillus niger CBS 513.88. Nature Biotechnol. 2007. V. 25 (2). P. 221–231.
Pinna D. Fungal physiology and the formation of calcium oxalate films on stone monuments. Aerobiologia. 1993. V. 9. P. 157–167.
Portnoy T., Margeot A., Linke R. et al. The CRE1 carbon catabolite repressor of the fungus Trichoderma reesei: a master regulator of carbon assimilation. BMC Genomics. 2011. V. 12. P. 269.
Posso E.J.S., de Prager M.S., Rojas C.A.C. Organic acids production by rhizosphere microorganisms isolated from a Typic Melanudands and its effects on the inorganic phosphates solubilization. Acta Agronomica J. 2017. V. 66 (2). P. 241–247. https://doi.org/10.15446/acag.v66n2.56148
Prabu R., Chand T., Raksha S. Improvement of Aspergillus niger for sodium gluconate synthesis by UV mutation method. J. Chemistry. 2012. V. 9 (4). P. 2052–2057. https://doi.org/10.1155/2012/768901
Prusky D., Lichter A. Mechanisms modulating fungal attack in post-harvest pathogen interactions and their control. Eur. J. Plant Pathol. 2008. 121. P. 281–289. https://doi.org/10.1007/s10658-007-9257-y
Prusky D., McEvoy J.L., Saftner R. et al. Relationship between host acidification and virulence of Penicillium spp. on apple and citrus fruit. Phytopathology. 2004. V. 94. P. 44–51. https://doi.org/10.1094/PHYTO.2004.94.1.44
Ramachandran S., Fontanille P., Pandey A. et al. Gluconic acid: a review. Food Technol. Biotechnol. 2006. V. 44. V. 2. P. 185–195.
Ramsay L.M., Sayer J.A., Gadd G.M. Stress responses of fungal colonies towards metals. In: The fungal colony. Cambridge University Press, Cambridge, 1999. P. 178–200.
Roehrl M.H., Croft W.J., Liao Q. et al. Hemorrhagic pulmonary oxalosis secondary to a noninvasive Aspergillus niger fungus ball. Virchows Arch. 2007. V. 451 (6). P. 1067–1073. https://doi.org/10.1007/s00428-007-0487-3
Rollins J.A., Dickman M.B. pH signaling in Sclerotinia sclerotiorum: identification of a pacC/RIM1 homolog. Appl. Environm. Microbiol. 2001. V. 67. P. 75–81. https://doi.org/10.1128/AEM.67.1.75-81.2001
Rosling A., Lindahl B.D., Taylor A.F.S. et al. Mycelial growth and substrate acidic cation of ectomycorrhizal fungi in response to diferent minerals. Microbiol. Ecol. 2004. V. 47. P. 31–37. https://doi.org/10.1016/S0168-6496(03)00222-8
Ruijter G.J., van de Vondervoort P.J.I., Visser J. Oxalic acid production by Aspergillus niger: An oxalate-non- producing mutant produces citric acid at pH 5 and in the presence of manganese. Microbiology. 1999. № 145. P. 2569–2576. https://doi.org/10.1099/00221287-145-9-2569
Ryan P.R., Delhaize E., Jones D.L. Function and mechanism of organic anion exudation from plant roots. Ann. Rev. Plant Physiol. Plant Molec. Biol. 2001. V. 52. P. 527–560. https://doi.org/10.1146/annurev.arplant.52.1.527
Rymowicz W., Lenart D. Comparison of different strains of Aspergillus niger for oxalic acid production from lipid substrates. Electronic journal of Polish agricultural universities. 2002. V. 7. http://www.ejpau.media.pl/volume7/issue2/biotechnology/abs-01.html
Sazanova K.V., Kirtsideli I.Yu. Influence of UV-irradiation on microfungi, isolated from antarctic habitats. Mikologiya i fitopatologiya. 2014. V. 48 (5). P. 315–321. (in Russ.).
Sazanova K., Osmolovskaya N., Schiparev S. et al. Organic acids induce tolerance to zinc- and copper-exposed fungi under various growth conditions. Current Microbiol. 2015. V. 70. P. 520–527. https://doi.org/10.1007/s00284-014-0751-0
Sazanova K.V., Vlasov D.Y., Osmolovskay N.G. et al. Significance and regulation of acids production by rock-inhabited fungi. In: Biogenic-abiogenic interactions in natural and anthropogenic systems. Lecture notes in Earth system sciences. Springer Nature, 2016, pp. 379–392. https://doi.org/10.1007/978-3-319-24987-2_29
Scheerer S., Ortega-Morales O., Gaylarde C. Microbial deterioration of stone monuments – An updated overview. Adv. Applied Microbiol. 2009. V. 66. P. 97–139. https://doi.org/10.1016/S0065-2164(08)00805-8
Schilling J.S., Jellison J. Oxalate regulation by two brown rot fungi decaying oxalate-amended and non-amended wood. Holzforschung. 2005. V. 59. P. 681–688. https://doi.org/10.1515/HF.2005.109
Schlosser D., Höfer C. Laccase-catalyzed oxidation of Mn2+ in the presence of natural Mn3+ chelators as a novel source of extracellular H2O2 production and its impact on manganese peroxidase. Appl. Environm. Microbiol. 2002. V. 68 (7). P. 3514–3521. https://doi.org/10.1128/AEM.68.7.3514-3521.2002
Schmalenberger A., Duran A.L., Bray A.W. et al. Oxalate secretion by ectomycorrhizal Paxillus involutus is mineral-specific and controls calcium weathering from minerals. Scientific Reports. 2015. V. 5 (12187). https://doi.org/10.1038/srep12187
Sharma T., Dreyer I., Kochian L. et al. The ALMT family of organic acid transporters in plants and their involvement in detoxification and nutrient security. Front. Plant Sci. 2016. V. 7 (1488). https://doi.org/10.3389/fpls.2016.01488
Show P.L., Oladele K.O., Siew Q.Y. et al. Overview of citric acid production from Aspergillus niger. Frontiers in Life Science. 2015. V. 8 (3). https://doi.org/10.1080/21553769.2015.1033653
Singh O.V., Sharma A., Singh R.P. Optimisation of fermentation conditions for gluconic acid production by a mutant of Aspergillus niger. Indian J. Experim. Biol. 2001. V. 39. P. 1136–1143.
Skory C.D. Isolation and expression of lactate dehydrogenase genes from Rhizopus oryzae. Appl. Environm. Microbiol. 2000. V. 66. P. 2343–2348. https://doi.org/10.1128/aem.66.6.2343-2348.2000
Smits M.M., Bonneville S., Benning L.G. et al. Plant-driven weathering of apatite – the role of an ectomycorrhizal fungus. Geobiology. 2012. V. 10. P. 445–456. https://doi.org/10.1111/j.1472-4669.2012.00331.x
Sterflinger K. Fungi: Their role in deterioration of cultural heritage. Fungal Biol. Rev. 2010. V. 24. P. 47–55. https://doi.org/10.1016/j.fbr.2010.03.003
Sturm E.V., Frank-Kamenetskaya O.V., Vlasov D.Yu. et al. Crystallization of calcium oxalate hydrates by interaction of calcite marble with fungus Aspergillus niger. American Mineralogist. 2015. V. 100. P. 2559–2565. https://doi.org/10.2138/am-2015-5104
Tembhurkar V.R., Joshi S.V., Dama L.B. et al. Random mutageneisis stimulated overproduction of citric acid by Aspergillus niger. DAV International J. Sci. 2012. V. 1. P. 53–55.
Vasanthabharathi V., Sajitha N., Jayalakshmi S. Citric acid production from U-V mutated estuarine Aspergillus niger. Advances in Biological Research. 2013. V. 7 (3). P. 89–94. https://doi.org/10.5829/idosi.abr.2013.7.3.73109
Vaughan D.J., Pattrick R.A.D., Wogelius R.A. Minerals, me-tals and molecules: ore and environmental mineralogy in the new millennium. Mineralogical Magazine. 2002. V. 66. P. 653–676.
Verhoeff K., Leeman M., Vanpeer R. et al. Changes in pH and the production of organic acids during colonization of tomato petioles by Botrytis cinerea. Journal of Phytopathology. 1988. V. 122. P. 327–336.
Vlasov D.Yu., Frank-Kamenetskaya O.V., Zelenskaya M.S. et al. In: Aspergillus niger. Pathogenicity, cultivation and uses. Ed. E. Baughan N.Y., 2020, p. 123.
Wallander H., Wickman T. Biotite and microcline as potassium sources in ectomycorrhizal and non-mycorrhizal Pinus sylvestris seedlings. Mycorrhiza. 1999. V. 9. P. 25–32.
Wang P., Wang T.-Y., Wu S.-H. et al. Effect of arbuscular mycorrhizal fungi on rhizosphere organic acid content and microbial activity of trifoliate orange under different low P conditions. Archives of Agronomy and Soil Sci. 2019. V. 65 (14). https://doi.org/10.1080/03650340.2019.1590555
Warscheid T., Braams J. Biodeterioration of stone: A review. Int. Biodeterior. Biodegradation. 2000. V. 46. P. 343–368. https://doi.org/10.1016/S0964-8305(00)00109-8
Watanabe T., Nobukazu S., Suzuki S. et al. Oxalate efflux transporter from the brown rot fungus Fomitopsis palustris. Appl. Environm. Microbiol. 2010. V. 76 (23). P. 7683–7690. https://doi.org/10.1128/AEM.00829-10
Williams B., Kabbage M., Kim H.J. et al. Tipping the balance: Sclerotinia sclerotiorum secreted oxalic acid suppresses host defenses by manipulating the host redox environment. PLoS Pathogens. 2011. V. 7. e1002107. https://doi.org/10.1371/journal.ppat.1002107
Williams L.E., Pittman J.K. Dissecting pathways involved in manganese homeostasis and stress in higher plant cells. Cell Biology of Metals and Nutrients. 2010. P. 95–117.
Willke T., Vorlop K.D. Biotechnological production of itaconic acid. Appl. Microbiol. Biotechnol. 2001. V. 56. P. 289–295. https://doi.org/10.1007/s002530100685
Woronick C.L., Johnson M.J. Carbon dioxide fixation by cell-free extracts of Aspergillus niger. J. Biol. Chem. 1960. V. 235. P. 9–15.
Xiao-ting C., Jie L., Xue-feng S. et al. The physiological and molecular responses of Arabidopsis thaliana to the stress of oxalic acid. Agricultural Sciences in China. 2009. V. 8 (7). P. 828–834. https://doi.org/10.1016/S1671-2927(08)60284-9
Xu B.D., Madrit C., Röhr M. et al. The influence of type and concentration of the carbon source on production of citric acid by Aspergillus niger. Appl. Microbiol. Biotechnol. 1989. V. 3. P. 553–558.
Yadav P., Chauhan A.K., Singh R.B. et al. Organic acids: microbial sources, production, and applications. In: Functional foods and nutraceuticals in metabolic and non-communicable diseases. Academic Press, 2022, pp. 325–337.
Yu R.C., Hang Y.D. Purification and characterization of NAD-dependent lactate dehydrogenase from Rhizopus oryzae. Food Chemistry. 1991. V. 41. P. 219–225. https://doi.org/10.1016/0308-8146(91)90044-O
Zhang A., Röhr M. Citric acid fermentation and heavy metal ions: II. The action of elevated manganese ion concentrations. Acta Biotechnol. 2002a. V. 22. P. 375–382. https://doi.org/10.1002/1521-3846(200207)22:3/4<375::AID-ABIO375>3.0.CO;2-Z
Zhang A., Röhr M. Effects of varied phosphorus concentrations on citric acid fermentation by Aspergillus niger. Acta Biotechnol. 2002b. V. 22. P. 383–390. https://doi.org/10.1002/1521-3846(200207)22:3/4<383::AID-ABIO383>3.0.CO;2-2
Zhang Z.Y., Bo J., Kelly J.M. Production of lactic acid from renewable materials by Rhizopus fungi. Biochemical Engineering J. 2007. V. 35. P. 251–263. https://doi.org/10.1016/j.bej.2007.01.028
Zhuravsky G.I. Gas exchange and the formation of citric acid in Aspergillus niger. Dr. Sci. Thesis. Leningrad, 1934.
Беннет-Кларк Т.А. (Bennet-Clark) Роль органических кислот в обмене веществ растений. Л.: Государственное издательство биологической и медицинской литературы, 1938. 130 с.
Буткевич В.С. (Butkevich) Избранные труды. М.: Изд-во Академии наук, 1957. 632 с.
Журавский Г.И. (Zhravsky) Газообмен и образование лимонной кислоты у Aspergillus niger // Дисc. … канд. биол. наук. Л., 1934. 112 с.
Сазанова К.В., Кирцидели И.Ю. (Sazanova, Kirtsideli) Влияние ультрафиолетового облучения на микроскопические грибы, изолированные из антарктических местообитаний. Микология и фитопатология. 2014. Т. 48. № 5. С. 315–321.
Дополнительные материалы отсутствуют.
Инструменты
Микология и фитопатология