Российский физиологический журнал им. И.М. Сеченова, 2020, T. 106, № 8, стр. 927-951
Biophotons and visual phenomena
V. I. Govardovskii *
Sechenov Institute of Evolutionary Physiology and Biochemistry Russion Academy of Sciences
Saint Petersburg, Russia
* E-mail: michael.firsov@gmail.com
Поступила в редакцию 13.06.2020
После доработки 23.06.2020
Принята к публикации 12.07.2020
Аннотация
The review considers modern un-orthodox ideas on the origin of visual phenomena of apparently various nature (photoreceptors’ dark light, negative afterimages, and various sorts of phosphenes such as electrophosphenes, magnetophosphenes, radiation phosphenes, and mechanophosphenes). The new framework provides a unifying explanation for all the phenomena that are suggested to be a perception of biophotons (ultra-weak photon emission, UPE) that are generated mostly by lipid peroxidation (LPO) during routine cellular metabolism. In the paper, the biochemical mechanism of biophotons’ generation is briefly explained. It is concluded that the outer segments of retinal photoreceptors provide an excellent substrate for LPO thus being a good candidate for UPE production. Experiments show that the retina in complete darkness indeed emits an extremely low level of bioluminescence. Yet its intensity is two orders of magnitude below the dark-adapted visual threshold, and over 100 times smaller than necessary to generate the photoreceptors’ dark noise. UPE also cannot be a source of afterimages because its intensity is far too low. Besides, the background light necessary to produce a negative afterimage is actually supplied by the ambient light that passes into the eye through closed lids. All other sorts of phosphenes attributed to UPE cannot be produced by biophotons since it is known for at least 200 years that the phosphenes are seen in daylight, that is, at the intensities billions of times brighter than the intensity of UPE. Thus, the new explanatory framework for all visual phenomena based on UPE should be discarded in its entirety.
The biophoton saga began in 1923–1924 when a Russian biologist Alexander Gurwitsch (sometimes spelled as Gurvich) discovered a non-contact interaction between two living tissue samples that were in the active mitotic state. A damaged and repairing area of frog skin, growing onion roots, or the cells of corneal epithelium induced mitoses in spatially separated pieces of the corresponding tissue. Further, it has been shown that the interaction is mediated by a sort of electromagnetic radiation, probably in the ultraviolet (UV) part of the spectrum. Gurwitsch called the radiation “the mitogenetic rays” and connected it to his previously conceived idea of a “mitogenetic field” that controls embryonic development [1–3].
There was a burst of research activity in the field during 1920–1950s aimed at designing methods of physical detection of the newly discovered radiation, and on studying its properties. The results were often disappointing, perhaps due to the insufficient sensitivity of light detectors available at that time. The history of confusions is described in detail in a recent review [4]. Finally, the entire field was qualified by Nobel physicist Langmuir as a “pathological science” ([5], original Langmuir’s lecture of 1953 transcribed and reprinted by Hall, 1989).
However, further development of high-sensitive photomultipliers working at liquid nitrogen temperature left no doubt about the existence of spontaneous weak photon emission from essentially any biological tissue. Modern cooled CCD cameras create images of biological objects in their own light, by accumulating pixels activated by single photons [6–9]. Thus the studies of the “ultra-weak photon emission” (UPE), or biophotons, form now a respectable (albeit inhomogeneous) field of research. UPE is also used now to provide a unifying explanation of various visual phenomena not related to each other, such as retinal “dark light,” afterimages, radiation phosphenes, electrophosphenes, magnetophosphenes, and mechanophosphenes. The presented review deals specifically with this field of research.
What is UPE?
There is no strict definition of the Ultraweak Photon Emission. The term mostly applies to any sort of light emission from living sources that is too weak to be seen with a dark-adapted human eye (Fig. 1). It is also tacitly assumed that biophotons are mostly produced as a byproduct of routine cellular oxidative metabolism. This, at least, clearly opposes the situations when biochemical machinery is specially designed to provide bright visible light that is used for orientation and signalization (e.g., various versions of luciferin-luciferase systems).
Fig. 1.
Range of UPE intensity compared with natural lighting conditions and range of human vision. Photon flux density is for 555-nm wavelength, at the maximum of human diurnal sensitivity.
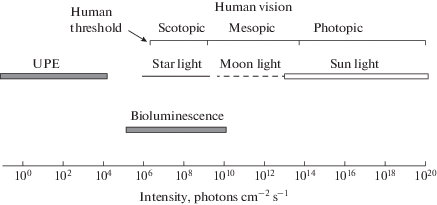
However, such a definition is a bit dubious. The same biochemistry, dependent on conditions, may probably generate the emission either barely detectable by best available sensors, or quite visible with a naked eye. On the other hand, it is often implied that UPE may serve various physiological functions within organisms, from the control of embryonic development (“mitogenetic rays”) to information transmission in the nervous system, and consciousness. Then the UPE should be produced quite purportedly in a controlled way rather than being a waste byproduct of metabolism. In these hypothetical scenarios, “ultra-weakness” may be either necessary or irrelevant, or even deteriorating to supposed functions.
Therefore, one should keep in mind that the term “UPE” or “biophotons” in the literature (and within this review) may have different meanings depending on the context.
Mechanisms of UPE generation
Biophotons are generated in reactions initiated by Reactive Oxygen Species (ROS). There are various sorts of ROSs and multiple mechanisms of their production, for instance, by UV light, ionizing electromagnetic radiation (X-rays and γ-rays), high-energy particles, and chemically induced oxidative stress. At this place, however, we are interested in mechanisms of ROS production by organisms in their normal functional state. The most important of them are listed in Table 1 [10].
Table 1.
Main ROS responsible for UPE
Electronically excited species | Emission wavelength |
---|---|
Triplet excited carbonyl | 350–560 nm |
Singlet excited pigment (melanin) | 360–560 nm |
Dimolar singlet oxygen | 634, 703 nm |
Monomolar singlet oxygen | 1270 nm |
Among them, the triplet excited carbonyls are the most intensely emitting in the visible part of the spectrum [10–12], so they are the most appropriate to produce visual phenomena.
Primary radicals are generated in routine cellular oxidative metabolism. There are numerous reviews on the topics. Some explanation of general principles with omitting technical details is given in [10, 13, 14]. A comprehensive review [15] also provides a historical background, including a significant body of Russian works.
Three of the most relevant intracellular sources of ROS are shown in Fig. 2.
Fig. 2.
Three principal sources of ROS in routine cellular metabolism (after [10]). A. Cit C, cytochrome C; UQ, ubiquinone; mt SOD, mitochondrial superoxide dismutase. B. AA, arachidonic acid; HPETE, arachidonic acid hydroperoxide, cSOD, cytoplasmic superoxide dismutase. C. NADPH-oxidase is a multi-subunit enzyme complex whose components are either transmembrane or cytosolic membrane-associated proteins.
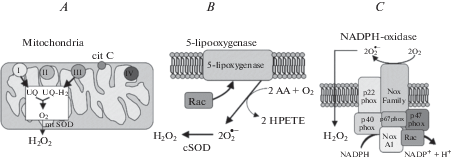
Mitochondria
At certain steps in the electron transport chain in mitochondria, approximately 1/20 of total electron flow is diverted from the ATP-forming pathway and produces superoxide anion $\left( {{\text{O}}_{{\text{2}}}^{{\centerdot - }}} \right).$ The latter is further converted by mitochondrial superoxide dismutase (mSOD) to hydrogen peroxide (H2O2), which can exit to the cytoplasm (Fig. 2A).
5-lipooxygenase
5-lipooxygenase (5-LOX) is normally membrane-associated enzyme that is involved in the synthesis of leukotrienes from arachidonic acid (AA). It oxidizes two AA, thus forming two superoxide anions, which are then converted to H2O2 by cytoplasmic superoxide dismutase (cSOD) (Fig. 2B).
NADPH oxidase
NADPH oxidase (NOX) is a transmembrane protein complex that catalyzes the production of superoxide radicals and further H2O2 by oxidizing NADPH with extracellular O2 (Fig. 2C). The set of NOX subunits is different in phagocytic- and non-phagocytic cells. Fig. 2C shows the composition of NOX in non-phagocytic cells.
In all the three cases, the same ROS, superoxide ${\text{O}}_{{\text{2}}}^{{\centerdot - }}$ is primarily formed. It is toxic, so within the cell, it is converted by SOD to hydrogen peroxide. H2O2 further yields superoxide anion ${\text{O}}{{{\text{H}}}^{\centerdot }}$ and hydroperoxyl radical ${\text{HO}}{{{\text{O}}}^{\centerdot }}$, which play a major role in lipid peroxidation and biophoton emission.
Lipid peroxidation and chemiluminescence
The primary source of biophoton emission in living tissues is lipid peroxidation [11, 12, 15, 16]. The free-radical lipid peroxidation is a chain reaction in which one radical can initiate the oxidation of many lipid molecules, mainly phospholipids containing polyunsaturated fatty acids (PUFA) (for a review see [16]).
PUFAs contain several double bonds in their carbon chain. In many, the cis-double bonds are separated from each other by a methylene bridge (–CH2–). The bridge contains two hydrogens that readily react with ROS, such as superoxide anion ${\text{O}}{{{\text{H}}}^{\centerdot }}$ and hydroperoxyl radical ${\text{HO}}{{{\text{O}}}^{\centerdot }}$. As a result, water and a fatty acid radical ${{{\text{L}}}^{\centerdot }}$ are formed (Fig. 3). The radical initiates a branched-chain reaction in which multiple copies of the initial radical (along with other products) are generated (propagation reactions, Fig. 4). The chain reaction terminates in a series of reactions when two radicals interact to form a non-radical product. One of the intermediates is a triplet excited carbonyl in the fatty acid chain that, when returning to the singlet state, emits visible photons (Fig. 4) [10–12].
Fig. 3.
Formation of lipid radicals by the interaction of a superoxide anion with a methylene bridge in PUFA.
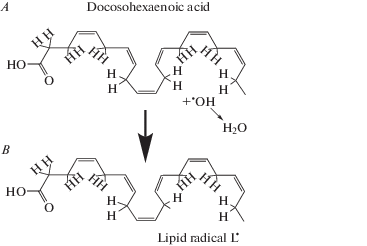
Fig. 4.
Branched-chain reactions of lipid peroxidation. In the initiation reaction (1), ROS oxidizes a lipid thus forming a lipid radical ${{{\text{L}}}^{\centerdot }}$. In propagation reaction (2), ${{{\text{L}}}^{\centerdot }}$ interacts with molecular oxygen and produces various forms of active lipids, including a copy of initial ${{{\text{L}}}^{\centerdot }}$, thus starting a new chain of oxidation (reactions 3, 4). The multiplication of chains terminates by a series of reactions when two radicals interact to yield non-radical products and a carbonyl instead of the methylene bridge in the fatty acid chain. The carbonyl is in the triplet excited state and transits to the singlet state emitting a visible photon.
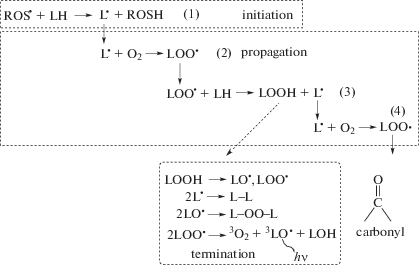
The retina contains a high concentration of PUFA, the highest of any vertebrate tissue [17]. Most of PUFAs is situated in outer segments of photoreceptor that consist of stacks of hundreds or thousands of membrane discs. Half of all acyl chains in phospholipids of the outer segments of photoreceptors belong to 22:6n-3 fatty acids, mainly docosahexaenoic acid (Fig. 3, 4) [18]. High level of oxidative metabolism in retinal rods and cones and a high content of PUFAs in their outer segments makes photoreceptors an excellent potential source of biophoton emission whose level could depend on the cells’ state. Combined with possible high sensitivity of rods and cones to their UPE, it makes the recent ideas that UPE can be a source of various visual phenomena worth considering [19–21].
Application of UPE to research, and suggested functions of biophotons
It is believed that in most cases, the UPE is produced as a byproduct of cellular metabolism (see above). Hence, starting from the first successful attempts to quantitatively measure the UPE, it has been used to study the underlying biochemical mechanisms. The experiments were often done in vitro on cell cultures and other bulk biochemical preparations. This provided a large amount of the sample (hence large signal) and allowed precise control of experimental conditions. Essentially all the available knowledge on the mechanisms of UPE production is gained from this sort of research (reviewed in [8, 15, 22]).
On the other hand, UPE can serve as a sensor to study metabolic processes and their alterations in normal and pathological conditions in vivo on the intact organism. Alexander Gurwitsch attempted to apply “mitogenic rays” for tumor diagnostics and was awarded in 1941 the Stalin prize supposedly for establishing the method at the governmental clinics in Moscow [23]. Whatever real were the early results, presently, it is hoped that modern high-sensitive techniques of UPE registration and imaging may provide a minimum-invasive diagnostic tool for clinical use. This is a vast area actively developing now [7, 8, 24–26].
Two above examples deal with the use of UPE as a research or diagnostic tool but say nothing on the possible role of UPE as a driving factor of the physiological functions of the biophoton-producing organisms. With the present surge in the number of poorly-reviewed (or apparently not reviewed at all) online publications, there is no shortage of ideas on what biophotons could do, or supposedly are proven doing, even in well-established areas of research with rich experimental and theoretical background. In this review, I will select just a small fraction of published examples of what I would call “reckless speculations.”
Biophotons are considered means of distant communication between organisms to transmit signals of danger or stress. It is reported that a bacterial culture placed in a light-tight metal box and chemically stressed by adding hydrogen peroxide changed the level of its fluorescence. In turn, the second culture placed in a similar light-tight box 5 meters apart responded by changing its bioemission [27]. UPE in the UV-A range is implied in a bystander effect between two groups of fish, one of which was X-ray irradiated. Remarkably, the effect induced in the intact group was observed even if the irradiation of the experimental group was scheduled for the next day [28].
Biophotons are suggested as a means for signal propagation in the nervous system, along with (or even instead of) well-established mechanisms of electrical and chemical transmission. Nerve fibers are supposed to be light guides conducting biophotons from a source somewhere in the neuron body towards targets in its output structure(s) [6, 29, 30].
The idea is supported by the fact that the intensity of UPE may correlate with the level of excitation in various parts of the brain [31]. Taking into account that UPE is mostly produced in routine cellular metabolism, one well may expect that any activity-related changes in brain metabolism would be reflected in UPE production. Yet this does not mean the reverse, that is, that changes in UPE are causing changes in neural activity.
The speculations on information processing by biophotons came to logical completeness in a new theory of vision. It has been suggested that perhaps the electrical responses of retinal photoreceptors and the following neurons may not be quite relevant to the visual function. According to new ideas, phototransduction in rods and cones may change cellular metabolism that, in turn, produces biophotons sent to the brain along with the light guides in the optic nerve. Further chain of alternating metabolic changes and biophoton production eventually leads to the creation of light replicas of observed objects somewhere in central brain structures [32, 33]. So the enigma of human vision is solved. The only remaining problem is where is situated the homunculus that observes the biophoton replica. But the problem may soon be solved within the emerging framework of consciousness and intelligence made of biophotons [34, 35].
I do not see how any meaningful discussion of the unorthodox ideas mentioned above is possible. However, the most promising application of biophotons could be to explain various visual phenomena. Indeed, there is a sub-field of less global theories on the role of UPE in specific aspects of vision that can be reasonably discussed in terms of available knowledge and subjected to experimental tests. The rest of the review will critically discuss the possible contribution of biophotons to retinal dark noise and visual phosphenes of various origins, based on recent experimental data on retinal UPE, and, often, on classical knowledge of the phenomena.
Retinal dark light and UPE
The concept of the retinal “dark light” dates back to at least the 19th century [36]. Even in complete darkness, an observer does not see something absolutely black, but rather “an irregular feebly illuminated field with numerous fluctuating spots of light” [36, p. 12–13]. The phenomenon was called the intrinsic light of the retina, or der Eigengrau in German. The plain experimental observations were soon incorporated into a theoretical framework. The famous Weber–Fechner relation (“sensation is proportional to the logarithm of stimulation”) was derived from psychophysical experiments on sensory thresholds. In an equivalent formulation, it states that the minimum perceptible increase in the intensity of stimulation ΔB is a constant fraction of the stimulus itself (B):
For vision, it essentially means the preservation of perceived contrasts in a scene independently of changes in the level of ambient illumination. Later it was found that the eq. 1 is inaccurate at low B, and a more appropriate relation is
(The history of the equations is described in [36], p. 170–190).
It looks like a certain weak signal Bd is present in the visual system, even in complete darkness (B = 0). This “dark light” from Equation (2) was associated with (but not necessarily identical to) the intrinsic retinal light.
It was supposed in the 1940–1950s that the sense of the dark light is produced in the retina by rare spontaneous activations of molecules of the visual pigment rhodopsin, without absorbing any photon [37–39]. The authors suggested that the activation happens due to random thermal transitions in rhodopsin and comprises an irreducible source of the visual noise. The noise could be the factor that limits the ultimate sensitivity of vision. Furthermore, it has been hypothesized that the noise level must depend on the spectral sensitivity of the photoreceptor [38, 39]. Visual pigments of red-sensitive cones need low quantum energy for activation, so they have an excellent chance to acquire it from thermal motion and produce “dark light.” More short-wave sensitive rod visual pigments need higher energy for activation, so they are less “noisy.” Crude calculations had shown that the difference in the photon energy at the peak sensitivity of rods and cones could explain the difference in sensitivity between human diurnal (cone-driven) and nocturnal (rod-driven) vision. This proposition is now known as “Horace Barlow’s hypothesis.”
It took over 20 years to experimentally prove the existence of rhodopsin-initiated dark noise in retinal photoreceptors. Suction-pipette recordings from single rods of the vertebrate retina have shown that in complete darkness, they produce random fluctuations of the flowing current (Fig. 5). The noise consisted of two components [40, 41]. The continuous noise is low-amplitude (few tenths of pA) oscillations that are symmetrical with respect to the dark level of the current. Its origin is still disputable [42–44]. The discrete noise consists of randomly occurring high-amplitude (in a few pA range) unipolar current waves. The waves are virtually identical to responses of the same rod to single photons (SPRs) [41, 43, 45] (Fig. 5B). First recorded from the rods of the toad, the SPR-like discrete noise was later detected in rods of any tested vertebrate species.
Fig. 5.
Continuous and discrete dark noise in a toad rod. A, continuous recording of the dark current. B, comparing the discrete dark events with the single-photon response of the same cell. Bold smooth line is an average of 40 responses to short flashes activating on average 1 R*. Superimposed is an average of 15 discrete dark events (mean ±1 SEM) that were cut off from the continuous trace in A. Recordings by Luba Astakhova.
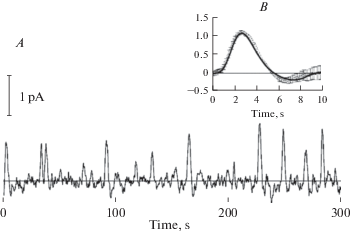
Vertebrate photoreceptors convert the absorption of a photon by a single rhodopsin molecule into an electrical response using a multistep amplification cascade. Each step involves hundreds of molecules of proteins and low molecular weight components; the inactivation of the cascade also needs many components (recent reviews [46–50]) (Fig. 6). It is quite improbable that such a complex chain of concerted events could be replicated by random fluctuations in the cascade unless it is initiated by a single molecule at its very beginning, that is, by activation of rhodopsin. Thus, the Barlow’s idea of activation of rhodopsin by internal thermal motion was generally accepted.
Fig. 6.
A simplified scheme of the phototransduction cascade in vertebrate rods and cones. Activation reactions are only shown. De-activation processes are omitted to avoid clutter. Light-activated rhodopsin R* interacts at the rate of about 100 s–1 with trimeric G-protein transducin and produces active transducin α-subunits Tα*-GTP. Each Tα*-GTP binds in the ratio 1 : 1 to cyclic GMP phosphodiesterase (PDE) and activates it. In turn, each PDE* hydrolyzes about 100 cGMP molecules per second. Concentration of cGMP decreases, and cGMP-controlled ionic channels of the plasma membrane close producing electrical response.
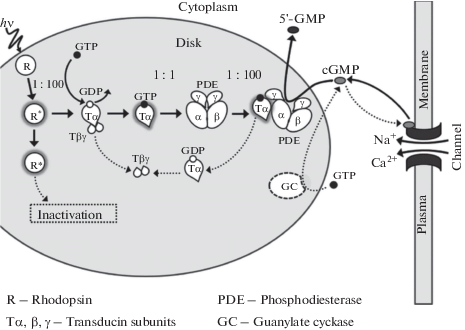
Barlow’s hypothesis was very fruitful because it could naturally explain important properties of vision, like different sensitivities of rods and cones, Purkinje shift, and lack of infrared vision in warm-blooded animals. It also predicted that there could be a trade-off between a spectral range of vision and thermal noise, so a sort of optimization of spectral sensitivity in accordance with the visual environment may be possible [51–54]. Yet, already, the first experimental confirmation of the existence of the dark noise revealed a serious problem. Accordingly to the hypothesis, a thermal motion was to provide enough energy to overcome the barrier between the ground and the light-exited states of rhodopsin (ΔEL). The barrier comprises 50 to 70 kcal/mol, depending on the position of the pigment’s absorbance maximum. However, the dependence of the rate of SPR-like events on temperature demonstrated far lower thermal activation energy ΔET ≈ 22 kcal/mol [41]. Also, Barlow’s formula predicts a very steep increase of the thermal noise with the red shift of the visual pigment absorbance, about 6000-fold per 50 nm. Actual dependence is far less steep, about 15-fold per the same shift (Fig. 7) [51–53].
The balance between the sensitivity to ambient light and the noise level might govern the adaptation of visual pigments to specific light environments [55]. Not surprisingly, a good deal of work has been done to resolve the above contradiction, to describe possible underlying intramolecular mechanisms theoretically, and to predict their impact on visual functions [56–58].
To reconcile the thermal noise hypothesis with the experimental data, one may assume that the isomerization of the 11-cis to all-trans rhodopsin chromophore by light and heat follows different routes. There is no trouble of thinking that the transition from 11-cis to all-trans configuration may not necessarily proceed via the (photo)excited state. The dark and activated basal states of the chromophore might be separated by an energy barrier lower than ΔEL, thus allowing smoother thermal transition. Robert Barlow with co-workers suggested that the transition follows via a de-protonated state of the chromophore that is supposed to be less stable than the protonated one [59, 60]. Under this assumption, the frequency of the SPR-like dark events should be strongly pH-dependent, increasing 10-fold per each unit of alkalization. The proposition was tested by measuring the frequency of dark events in Limulus photoreceptors at different pHs and indeed was supported by the data [59]. Unfortunately, a similar test on vertebrate photoreceptors showed no effect on pH on the discrete dark noise [54, 61].
Also, the function of Noise vs. Wavelength of maximum absorption is not unique. A few measurements on long-wave sensitive cone visual pigments expressed in rods yielded the noise level two orders of magnitude higher than predicted (and demonstrated) for rod pigments with the same absorbance maximum [62] (Fig. 7).
Understanding that the early model of thermal excitation based on Boltzmann statistics is an apparent oversimplification lead to the creation of more sophisticated approaches that included attempts to compute the transitions in rhodopsin ab initio on supercomputers. The present situation is discussed in [51]. It can be summarized as follows: crude predictions of existing simple theories are in general agreement with the experiment. Yet one should humbly admit that a good molecular theory of the thermal noise is still lacking. This does not mean, however, that the idea itself is wrong.
A radical solution to the problem was proposed a few years ago. It was suggested that rhodopsin molecules are activated not by heat but rather by real photons from UPE [63–67]. The reasoning is that the retina is one of the most metabolically active tissues in the organism, so it must be a good source of ROS. Besides, outer segments of rods and cones contain a high amount of polyunsaturated fatty acids, thus providing an excellent substrate for UPE production. There is no functional theory (actually, no theory et al.) behind the hypothesis, but it has certain experimental support. All parts of the eye (cornea, vitreous body, and retina of rat and frog) were studied with a high-sensitive cooled CCD camera and shown to emit biophotons [64, 65].
Properties of the discrete noise in rods are well known, so the source of biophoton emission (if it is responsible for the noise) can be pinpointed with confidence since the discrete noise can be recorded both from rods attached to the retina and from solitary isolated cells (for instance [41, 43], the biophotons must be produced by photoreceptors themselves. Moreover, discrete noise is also recorded from detached rod outer segments provided that necessary metabolites are supplied via the perfusion solution [42]. Hence the primary source of biophotons should be the oxidation of polyunsaturated fatty acids of the photoreceptor membranes.
It has been suggested that the biophoton hypothesis can explain the high noise level in cones since cones are supposed to have bigger ellipsoids hence more active metabolism [67]. The argument is flawed for two reasons. Firstly, tightly-packed rods and cones within the retina share the same UPE pool. Secondly, cones comprise just a small fraction of photoreceptors (e.g., one cone per 20 to 50 rods in mammalian retinas). Therefore, no significant difference in noise level between rods and cones is expected. Critical for the biophoton hypothesis, human red cone visual pigment produces high discrete dark noise when expressed in rods along with their native rhodopsin [62]. High noise in red-sensitive pigment compared to rhodopsin (Fig. 7) could be explained in favor of the biophoton hypothesis by assuming a specific spectral composition of photoreceptors’ UPE. If the maximum intensity of UPE were in the long-wave (red) end of the spectrum, it would excite cones far more efficiently than rods. No real data on the spectrum of retinal UPE is available, but further experiments made this suggestion improbable (see below).
A precise quantitative test of the biophoton hypothesis is possible. The intensity of biophoton emission necessary to produce the dark noise can be calculated from physiological measurements and compared with the detected UPE level. Proper experiments were done on rods of frog Rana ridibunda and fish (sterlet sturgeon Acipenser ruthenus) [68]. The dark noise level was measured with the suction recordings from solitary isolated rods. UPE was registered from eyecups or pieces of the isolated perfused retina by a high-sensitive, broad waveband (200 to 800 nm) photomultiplier (PMT Hamamatsu R9110). Special attention was paid to establishing the quantitative relation between the level of rhodopsin activation and the intensity of UPE reaching the PMT (if UPE were to produce the observed dark noise). To do this, the retina in the experimental chamber was illuminated with a separate light source whose intensity was calibrated in situ in the same chamber by measuring rhodopsin bleaching. Further, propagation of light within the measuring system was computationally traced to estimate the ratios of the lights produced, absorbed within the rod outer segment, and reaching the PMT. In these experiments, retinal samples indeed generated measurable UPE [68]. Importantly, its level was the same as detected earlier with the cooled SSD camera by Li & Dai [65]. However, the intensity of the UPE was over 100 times lower than necessary to produce discrete dark noise.
The discrepancy between necessary and available light was estimated in [68] under the assumption that the spectrum of UPE is similar to the spectrum of the LED used for intensity calibration (peak at 505 nm, close to the maximum sensitivity of rods). If the UPE were more long-wave, its efficiency to produce dark noise in rods was lower, but in cones, it was higher. Specifically, the 1000-fold difference in cone and rod noise levels can be explained by different spectral sensitivities of their visual pigments combined with the biophoton spectrum peaking in red at 640–650 nm [68]. In this case, the same red UPE would excite red cones ∼1.000 times more efficiently than rods, in accordance with experimental observations [62]. Yet, to produce proper absolute noise levels in rods and cones, biophoton emission should then be ∼1.000 times greater than the equivalent rod-activating light at 505 nm. Since spectral sensitivity of the measuring system in [68] was flat, the corresponding PMT response to the rod- and cone-activating light should be 1.000 times more potent than shown in Fig. 8 (solid line). This would bring the discrepancy between the biophoton hypothesis and experiment to nearly five orders of magnitude.
Fig. 8.
Comparing biophoton emission with the rate of dark rhodopsin activation derived from electrophysiological recordings. Frog retinas. The dots with error bars are the average bioemission intensities (15 retinas, mean ±1 SEM). The high-amplitude black trace shows the bioemission signal that would produce the actual frequency of discrete dark waves (after [68]).
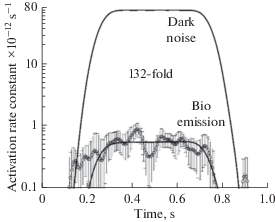
In sum, retina indeed produces reliably measurable UPE, but its intensity is over 100-fold lower than necessary to explain the photoreceptor dark noise. Thus, the biophoton hypothesis on the origin of retinal dark light must be rejected, and further experimental and theoretical work on the model of thermal activation of rhodopsin retains its importance.
Biophotons and afterimages
If one looks for a while at a bright source of light and then turns the light off or shuts eyes, a bright imprint of the source is seen for a certain time, slowly fading away. This is a positive afterimage. When the positive image fades completely, it may convert to its dark copy against a bleak retinal background. This is a negative afterimage. If the stimulus was achromatic, the afterimage is also achromatic. If the stimulus was colored, the afterimage appears in complementary colors (Cyan vs. Red, Magenta vs. Green, Yellow vs. Blue). There is a combination of three factors that are responsible for afterimages. Firstly, there is decaying signaling in retinal and central neural circuits that lasts for a while after the light was turned off. It forms a positive afterimage. The second is the loss of sensitivity in the illuminated area due to light adaptation. The third is a certain source of excitation of the entire retina that creates a light background. Then the light-adapted, low-sensitive previously illuminated area would look dark against the background. It creates a negative afterimage.
All three factors have mostly the retinal origin. If the transmission from the eye to the brain is blocked during the action of the inducing stimulus (for instance, by pressing the eyeball), the afterimage gets visible after the block is released [69]. On the other hand, specific features of the afterimage (color, duration, sharpness, dependence on visual surround, etc.) are affected by central mechanisms [70–72]. There is no shortage of the sites in the visual system where the signal persists, and sensitivity remains reduced after turning-off the inducing stimulus. It has recently been shown that two necessary components of afterimage, lasting signaling and post-stimulus desensitization, can be found already at the level of retinal rods at entirely physiological intensities of light [73].
The remaining problem is, what is the source of light background that is necessary to see a negative afterimage? As formulated by proponents of biophoton theories: “What is it, when our eyes are closed, that produces the long-lasting signal that makes static afterimages within our eyes interpreted by the brain as a visual experience?” [67, p. 111]. Their answer is, the stimulus-induced delayed secondary UPE.
It is supposed that the inducing stimulus changes photoreceptor metabolism, which, in turn, evokes secondary UPE from the retina. Thus, UPE from a formerly brightly stimulated area illuminates the entire eye from inside. This is the source of light background that is necessary to see a negative afterimage even with shut eyes [67]. The secondary UPE does exist indeed. Excised rat eye, lens, vitreous body, and retina steadily emit biophotons in complete darkness [64]. Bright illumination of the sample induces a transient increase in the intensity of the emission that lasts for a few minutes. Supposedly, this is the source of the full-field background that creates a negative afterimage.
The problem with the explanation is that, as shown in the previous section, the intensity of dark UPE from the retina is over two orders of magnitude below the visual threshold [68]. The induced surplus from delayed emission is an extra 20- to 100-times weaker (Fig. 1 in [64]). So the biophoton hypothesis of the afterimage must be rejected, too.
Curiously, there is no problem to solve at all. One needs not a light source within the eye to see negative afterimages. If the inducing stimulus is delivered in complete darkness, the only positive afterimage is seen. After it fades away, no negative afterimage appears. One can test it by first looking at the screen of one’s smartphone in a dark room, and then turning the smartphone off. If instead of turning the screen off, one simply shuts eyes, the positive afterimage will soon turn negative. Then, keeping eyes closed and covering the shining screen with one’s palm, one can repeatedly switch between positive and negative afterimages. “What is it, when our eyes are closed, that produces the long-lasting signal that makes static afterimages within our eyes interpreted by the brain as a visual experience?” It is the light that passes through our tightly shut lids. It is that simple.
Biophotons and phosphenes
The term “phosphenes” may refer to a wide range of phenomena when an observer sees the light without any apparent light source.
Seeing invisible light: limits of the human visible spectrum
It is commonly accepted that humans can perceive as light the electromagnetic radiation of wavelengths between 380 and 700 nm. The invisible radiation with λ < 380 nm is classified as ultraviolet (UV), and with λ > 700 nm, as infrared (IR). The limits are rather arbitrary and are set to correspond to the ends of the colorful spectral band, which is created from the sunlight by a prism or a diffraction grating. There is no sharp IR limit. Sensitivity to the long-wave radiation decreases roughly exponentially with increased wavelengths (reviewed in [74]). The IR light at 1050 nm can be seen by a dark-adapted eye if its intensity is 12.5 decimal log units above the rod visual threshold at 507 nm [75, 76]. A further shift to IR is possible with increased intensity and is only limited by the danger of vitreous and retinal heat damage.
The UV limit in humans is set by the transmittance of ocular media (cornea, lens, vitreous, and the retina itself). They are of yellow(ish) color and block the UV light from reaching photoreceptors. The yellowness grows with age soG that the UV border can be markedly different for young and elderly persons. The intrinsic sensitivity of retinal photoreceptors is substantially wider than is routinely measured in physiological experiments. It is formed by the absorbance spectrum of the corresponding visual pigment. The spectrum consists of three bands of interest (Fig. 10). The most prominent is the α-band whose peak in various pigments may lie between 350 and 630 nm (traditionally “visible” light) (reviewed in [74]).
The β-band of rhodopsin absorbance supports sensitivity to near UV between 400 and 300 nm (UVA). Quantum yield of rhodopsin activation in the β-band is equal to that in α‑band. So, the sensitivity at 350 nm is about 25% of maximum at 500–550 nm, which is substantially higher than in the officially “visible” red part of the spectrum (≥630 nm). In many vertebrate species, the eye media are transparent down to ≈300 nm. Therefore, a grass frog can see UVA invisible to humans [77].
The γ-band at 280 nm (UVB) is due to the absorption of light by aromatic amino acids (mostly by tryptophan and tyrosine) of the opsin moiety. Illumination with UVB of the rhodopsin solution in detergent bleaches it, forming the standard product all-trans-retinal [78]. Thus bleaching by UVB follows via the same route of 11-cis to all-trans isomerization of chromophore as initiated by α-band and β-band absorption and could start phototransduction. Frog rods show a peak of sensitivity at 280 nm, which is even higher than in the β-band [77] (dashed line in Figure 10). Quantum yield of rhodopsin bleaching and transduction activation at 280 nm is about 2.6 times lower than in the α-band and β-band [77, 78]. This reflects a relatively low efficiency of the intramolecular energy migration to the chromophore from aromatic amino acids that partly form the chromophore pocket.
The sensitivity in the γ-band cannot be of any behavioral importance, though since the intensity of UVB radiation in the natural environment is extremely low; besides, it is strongly absorbed by all proteins in the light path through the eye to photoreceptors.
Thus the vertebrate vision in the spectral band from near IR to UVB is supported by a single molecular mechanism, light-induced isomerization of the rhodopsin chromophore, with subsequent activation of the phototransduction cascade. The situation may change at an even shorter wavelength (higher photon energies) of X-rays and gamma-rays.
Visibility of X-rays
When Röntgen in 1895 discovered X-rays, he called them “invisible radiation.” However, other researchers soon found that the X-rays can produce a visual sensation in a dark-adapted observer. It was characterized as a faint greenish-bluish glow filling the eye. Rather soon, it has been shown that the sensation originates from the excitation of the retina rather than from possible X-ray-induced fluorescence of the eye media. The early studies on the properties and mechanisms of the generation of the X-ray phosphenes are summarized in [79, 80].
Apparently, the first electrophysiological study of the retinal response to X-rays was done by Lipetz [79], who registered spike responses from retinal ganglion cells of the frog eye. A parallel study of responses to light and X-ray stimulation revealed a similarity between their properties. Threshold intensities were determined, and the ability of X-rays to bleach rhodopsin in the retina was tested. The degree of rhodopsin bleaching was estimated without any quantitative method, though, just by visual inspection of the retinas’ color. It appeared that the ratio of threshold intensities for electrical responses and the intensities producing noticeable bleach was similar, of about 1 × 108, for both stimuli. The general conclusion was that the visual effects of light and X-rays are produced by their action on rhodopsin. However, the estimate of the threshold-to-bleach range was quite crude, with a possible error of an order of magnitude. Besides, bleaching doses of X-rays resulted in the denaturation of proteins as well. So the work did not prove that the physiological response to X-rays is caused by the isomerization of the rhodopsin chromophore, as in the case of visible light.
Later works relied on recordings of electroretinogram (ERG) and visually evoked cortical potentials. They showed that indeed the primary target for X-rays in the retina is a visual pigment, rhodopsin. At moderate intensities, ERG in response to X-rays and light were of virtually identical shapes, and their Response vs. Intensity curves were approximately congruent [80–83]. Importantly, at low intensities, ERG to both stimuli consisted of a unipolar cornea-positive b-wave; above a certain threshold intensity level, the b-wave was preceded by a brief cornea-negative a-wave which is known to reflect the electrical activity of photoreceptors. The electrical response of rods and cones is produced by a complex biochemical cascade started by cis-trans isomerization of rhodopsin chromophore (Fig. 6). Hence, the X-ray ERG was initiated by the action of X-rays on rhodopsin in photoreceptors rather than by their possible effect on secondary retinal neurons.
Also, X-rays bleached rhodopsin, both when applied to the living animals [84] and detergent extracts of the visual pigment [82, 84]. As already noticed by [80], the X-ray intensity that bleached a noticeable fraction of rhodopsin was 7 to 8 orders of magnitude higher than the dose necessary to evoke a threshold ERG. This is essentially what occurs with light stimulation, too. A threshold light ERG can be elicited by bleaching a single rhodopsin per rod, which may contain from 108 to 3 × 109 “dark” (unbleached) rhodopsins. The exact comparison of rhodopsin bleaching by light and X-rays is hampered by the fact that the doses of X-rays necessary for measurable bleach also damage the retina and denature proteins, including rhodopsin itself [80, 84]. Thus, the loss of Rh peak absorbance at 500 nm after high X-ray exposure partly reflects rhodopsin damage rather than its photoactivation [80, 84, 85]. Nevertheless, a substantial fraction of rhodopsin is converted to its physiologically active conformation, metarhodopsin II, which is evidenced by the appearance of meta II absorbance hump at 380 nm [85].
Cones also contribute to X-ray sensation. It is reported that the close-to-threshold bluish-green X-ray phosphenes in humans change their color to yellowish-green at high doses [79]. However, X-ray ERG was not recorded from a pure cone retina of a lizard Phrynosoma (horned toad) [82]. It seems that at least some cone visual pigments are less sensitive to X‑rays than rod rhodopsin. Along with generally lower sensitivity of cones compared to rods, lower efficiency of excitation of cone visual pigments by X-rays may prevent the detection of cone contribution to ERG in a pure cone or mixed rod-cone retinas.
One may conclude that X-rays of commonly used energies of quanta from 50 to 100 keV excite retinal rods in the same manner as the visible light does, that is, by isomerizing the rhodopsin chromophore. Various side effects caused by protein denaturation and possible direct action on retinal neurons at high doses cannot be excluded.
Phosphenes from high-energy particles
Flashes of light seen as a wide-field glow or bright spots, and sometimes interrupted streaks were reported by astronauts during the first long-lasting space flights [86–89]; recent reviews [90, 91]. Similar phosphenes are seen by patients with ocular and brain tumors that receive treatment with high-energy particles from an accelerator [92, 93]. The practical importance of accessing space travel radiation hazard and the risk of radiation damage in medical treatment provoked a good number of works on identifying the source(s) of the phenomenon [94–97]. Volunteers were presented with very low doses of accelerated particles of various sorts (typically just a few particles per trial) and reported their sensations. It appeared that bright light streaks could be produced by high-energy neutrons, alpha-particles, protons, pions, muons, nitrogen nuclei, and carbon ions (review [98]). The light streaks were seen at particles’ energies both below and above the Cerenkov radiation threshold. Diffuse flashes and glow only appeared if the energy of a (charged) particle exceeded the Cerenkov threshold.
There is a single work showing that low doses of deuterons with the energy of 15 MeV (below Cerenkov threshold) evoke ERG from the isolated retina of the frog [99]. Properties of the deuteron-induced and light-induced ERGs were similar. Particle ERG showed a distinct a-wave indicating activation of photoreceptors via a routine light-induced way, that is, by cis-trans isomerization of the chromophore. The authors also demonstrated the loss of rhodopsin peak at high irradiation levels. Remarkably, deuteron doses necessary to bleach a significant amount (≈50%) of rhodopsin appeared about nine orders of magnitude higher than the doses that evoked a threshold ERG. High doses also denatured rhodopsin. Unfortunately, the formation of the light-activated rhodopsin state (Metarhodopsin II) by high irradiation doses has not been tested in [99]. So, it remains unclear what fraction of rhodopsin is gone by denaturation, and what fraction was used for activation of visual signaling. Similar results were obtained with irradiation by α-particles with energies up to 30 MeV [99].
The results [99] have an important implication for the biophoton hypothesis of the production of radiation phosphenes. If rhodopsin were bleached by biophotons of whatever origin, one should expect a pretty high level of visible luminescence from the rhodopsin samples during irradiation. It is easy to calculate that bleaching 50% rhodopsin by the light needs illumination with 1000 lux during about 50 s that hardly qualifies as UPE. Therefore, if rhodopsin in chemiluminescence [100] or particle irradiation experiments [99, 101] were bleached by biophotons, the sample should shine like a torch. Yet in [99], a photomultiplier was used for testing the possible retinal luminescence during particle irradiation and detected nothing. Thus, at least in case of deuteron- or α-particle-evoked phosphenes, the biophoton hypothesis can be discarded.
There is only a couple of papers on the ability of UPE-type chemiluminescence or heavy particles to produce massive rhodopsin activation detectable by standard biochemical methods [100, 101]. In the first work [100], the authors demonstrated that the chemiluminescence from a xanthine/xanthine oxidase system with lipids and polyunsaturated fatty acids could bleach rhodopsin. We know since 1876 [102] that visible light bleaches rhodopsin, so the result of [100] is hardly surprising. The same group also reported that irradiation by 200 MeV 12C nuclei bleaches rhodopsin in the suspension of rod outer segments (RdOS), with the formation of Meta II-like product(s). Bleaching was supposedly caused by biophotons formed by RdOS lipid peroxidation with ROS created by 12C irradiation [101]. The conclusion, however, is in no way supported by the presented data. The results of 12C-bleaching experiments are illustrated by Fig. 1 in [101], which is just an exact duplicate of Fig. 3 from [100], where it demonstrates bleaching with visible light. Besides, no Meta II at all could be found in 12C irradiation experiments in using [101] protocol. The mammalian Meta II vanishes in about 5 min after formation at 37°C, by decaying to all-trans-retinal and opsin [103–105]. In [101], the irradiated samples were heated for 45 min at 60°C prior analysis (p. 3 in [101]), so no trace of Meta II would remain even if it were formed during irradiation. Thus, the solely available candidate for experimental support for the biophoton hypothesis of the origin of radiation phosphenes fails.
It seems that no real data is supporting the idea that the radiation phosphenes of whatever origin are caused by biophotons generated by lipid peroxidation via radiation-produced ROSs. At low exposures, any sort of ionizing radiation can activate rhodopsin either by real light via the Cerenkov mechanism or by energizing the rhodopsin molecule (and possibly its surround) with the following intramolecular energy transfer to the chromophore. High exposures result in tissue damage that obscures other possible effects of radiation. A portion of ionization products may generate biophotons, as suggested in [67, 100, 101]. Yet there is no experimental proof of the idea.
The author of the review dares to suggest a quite simple experiment that can clarify the situation. As mentioned above, marked rhodopsin loss by chromophore activation and further bleaching needs a substantial amount of visible light. Therefore, if rhodopsin in chemiluminescence [100] or particle irradiation experiments [101] were bleached by biophotons, the sample should shine brightly. It would be worth repeating the experiments [100, 101] using a physical light meter. No super-sensitive device is necessary. A household-rate instrument may suffice to settle the problem. Looking with a naked eye (if possible at a particle accelerator output) would also help.
Electro- and magneto-phosphenes
The light sensation can also be produced with electric or magnetic field stimulation of the retina or visual cortex. Here, the phosphenes of the eye origin are only considered. First observations of electric phosphenes were made already in the first half of the 19th century, in the general context of studying effects on electricity on human body. Various sources of the electric current were used (e.g., discharges from a Leyden jar or first galvanic batteries). The position of stimulating electrodes (e.g., on the forehead and the forearm) usually did not allow locating the target (the eye or the brain). In a rare experiment with local stimulation, Purkinje described phosphenes with a wire electrode placed on the cornea between shut lids. The early results were summarized by Helmholtz (p. 13–17 in [36]) and they are NOT of only historical interest now.
Apparently, first quantitative experiments that allowed determining the stimulated structures and phosphene thresholds were done in 1955 by Brindley [106] who used active electrodes pressed to conjunctiva at various places outside cornea, and the reference electrode in the mouth. As a stimulus, the alternate 50-Hz current was applied to a dark-adapted or moderately light-adapted eye. Currents near 20 μA were sufficient to evoke supra-threshold phosphenes.
Further works were aimed at discriminating between two probable targets for the current, optic nerve and the retina proper. Spatial distribution of phosphenes within the visual field with electrodes of various shapes and positions led to the conclusion that the phosphenes are produced in the retina when it is excited by the radial current, that is, by the current flowing perpendicular to the retinal surface. Tangential currents (flowing parallel to the retinal surface) that are supposed to activate axons of ganglion cells proved to be ineffective [106].
The modern biophoton hypothesis suggests that trans-retinal currents somehow change the metabolic processes in retinal cells, thus resulting in the production of extra UPE and phosphenes [63, 67]. For assessing the idea, important are BOTH early (19th century) and later (between 1960 and 1990) literature on the visual appearance of phosphenes themselves and their comparison with the responses to light stimulation in light- and dark-adapted states of the eye. It appeared that electrophosphenes could be evoked equally well in a dark-adapted and light-adapted state [36, 107, 108] and even against a light background that is equivalent to looking at a white surface illuminated with ≈7000 lux [108]. Thus, strong light adaptation does not substantially affect electrophosphenes. In turn, electrical phosphenes do not modify the adaptational state of the retina [107]. Supposed biophoton emission that could override light stimuli of daylight brightness can hardly be called UPE. Moreover, such current-evoked emission should make the eye brightly shining. Nothing of this sort was observed [36], so the suggested biophoton mechanism of electrophosphenes must be discarded.
Magnetophosphenes
A rather strong changing magnetic field can also induce phosphenes. The magnetophosphenes are usually described as a weak flickering light, colorless or slightly blue. The threshold for the 30-Hz alternating field was near 10 mT [109] and only changed somewhat between the dark-adapted state and rather strong background illumination (130 cd/m2). It has been concluded that magnetophosphenes could actually be electrophosphenes evoked by currents in the retina, which, in turn, are induced by an alternating magnetic field.
Attwell [110] provided a detailed theoretical analysis of currents and voltages generated by an alternating electromagnetic field in biological tissues. The estimate of the electric fields in a tissue at a magnetophosphene threshold was between 10 and 60 mV/m, which translates into 1 to 10 μV per photoreceptor length. This is close to the threshold for electrophosphenes (about 15 μV, see below). Some data, though, do not allow us to identify with certainty the magnetophosphenes as electrophosphenes produced by eddy currents. It was possible to induce magnetophosphenes in a person totally blind due to an advanced stage of retinitis pigmentosa, whose photoreceptor cells degenerated completely, supposedly losing their electrosensory structures, rod and cone synaptic terminals. This suggests that there were other partially preserved electrosensitive cells (perhaps bipolars and ganglion cells) in this observer [109].
Fig. 9.
A hypothetical scheme of production of phosphenes by biophotons generated by lipid peroxidation in photoreceptor outer segments. Various non-visual external stimuli (ionizing electromagnetic radiation, high-energy charged particles, electric current, oscillating magnetic field, and mechanical pressure on the eyeball) are supposed to change the photoreceptor metabolism thus changing the level of ROS generation and biophoton emission. After [63, 64, 67].
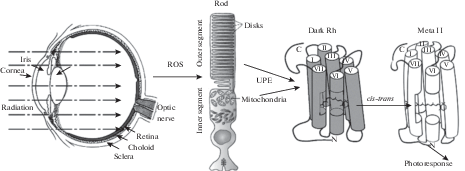
Fig. 10.
Between 250 and 650 nm, rhodopsin has three absorbance bands peaking at ≈ 500 (α), 350 (β), and 280 (γ) nm (solid line). The sensitivity of a rod in visible (α) and UV-A (β) band exactly coincides with the absorbance spectrum. Sensitivity in the γ-band is just 0.45 of the absorbance due to lower efficiency of energy transfer from aromatic amino acids to the chromophore (dashed line) [77, 78].
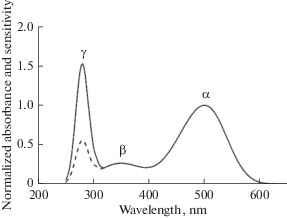
The visibility of magnetophosphenes against 130 cd/m2 light background [109] completely excludes the contribution of biophotons to the phenomenon. This background is about seven orders of magnitude above the rod visual threshold, and hence nine orders of magnitude above the resting biophoton emission from the retina [65, 68]. If biophotons were responsible for magnetophosphenes, it would imply a billion-fold surge of ROS production in the retina under modest magnetic stimulation.
Possible electrosensitive structures in the retina responsible for the production of electro- and magnetophosphene
It would be interesting to tentatively identify electrosensitive structures in the retina that are responsible for the production of electro- and magnetophosphenes. Since the phosphenes are evoked by the radial current, the most likely targets are radially orientated retinal cells. These are photoreceptor cells, bipolar cells, and radial (Müller) fibers. Photoreceptor cells, both rods and cones, have the unique organization of their output synapses. Structurally, the photoreceptor synapses contain synaptic ribbons, specific dense double-membrane structures that are surrounded by synaptic vesicles (Fig. 11) (rev. [111]). Functionally, they are drastically different from “ordinary” neuro-muscular junctions (NMJ) or axo-dendritic synapses in the rest of the nervous system. Typically, at the NMJ in a resting state, the presynaptic membrane has a high negative potential. Therefore, the transmitter release trough the membrane is slow if not absent altogether. The action potential that comes along the axon and reaches the terminal is a rather brief (a few milliseconds) but it provides a high-amplitude (a few tens millivolt) depolarization. It evokes a burst of powerful neurotransmitter release, which robustly excites the postsynaptic cell, mainly following the rule of All-Or-Nothing.
Fig. 11.
Small fragment of a synaptic terminal in the chicken cone. The presynaptic cone cytoplasm with a synaptic ribbon (arrow) is in the upper right corner; numerous synaptic vesicles containing glutamate are marked by small triangles. HC1 and HC2 are input dendrites of two horizontal cells. BC is an input dendrite of a bipolar.
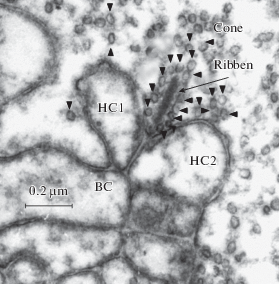
In contrast, at the ribbon synapse, the presynaptic membrane is substantially depolarized at rest. Therefore, the transmitter is continuously released from the presynaptic cell, at the rate that can be modulated either way by small changes in the membrane potential. The release smoothly decreases at hyperpolarization and increases at depolarization. Correspondingly, the membrane of a postsynaptic neuron can be gradually hyperpolarized or depolarized, thus coding the input in an analog manner.
Ribbon synapses are almost unique for sensory cells. Besides retinal photoreceptors, they are exploited by retinal bipolars, mechanoreceptors of the lateral line organs, and their evolutionary derivatives that include the organ of Corti and equilibrium receptors of the inner ear. In all cases, glutamate is used as an excitatory synaptic transmitter. Due to analog coding at the synapse, mechanosensory cells produce either hyperpolarizing or depolarizing postsynaptic response, dependent on the direction of deformation of their sensory cilia [111]. The two-way analog coding essentially means that there is no built-in sensory threshold, and the ability to detect weak stimuli is mostly limited by the ability to separate the useful signal from always present background noise. The utmost sensitivity is achieved in electroreceptors of ampules of Lorenzini in elasmobranch fish (sharks and rays). These organs consist of a small (1–2 mm) cysts supplied by long channels that run under the skin. The channels connect the cysts’ interior to various distant points on the surface of the fish body. The inner wall of the cyst is covered by sensory cells that essentially are holders for ribbon synapses. A few microvolt voltage applied to the ampule is sufficient to change the frequency of spikes in the nerve fibers of the ampullary nerve [112, 113]. The sign of the spike reaction (excitatory or inhibitory) depends on the polarity of the stimulus. In behavioral experiments, a shark reacted to electric fields as small as 5 nV/cm in water [112]. This corresponds to 0.05–0.1 μV voltage across the electroreceptive epithelium.
Clearly visible electrophosphenes can be evoked by the current of about 20 μA applied to cornea [106]. Calculations show that this current would produce a voltage change of ≈15 μV at the photoreceptor presynaptic membrane. The value is at least two orders of magnitude higher than the sensory threshold of Lorezinian ampules, so it certainly allows direct action of the current on photoreceptor ribbon synapse, to make phosphenes. Yet the value must better be compared with presynaptic responses in rods and cones at threshold light stimulation. Single-photon response (SPR) in human rods is near 1 mV [114] which is about two orders of magnitude higher than the threshold potential for the electrophosphenes. This doubts the ability of the direct electrical stimulation of the eye in situ to produce phosphenes via the rod pathway in the retina.
On the other hand, the SPR amplitude in a cone is about 15 μV [114], that is exactly what is necessary to produce electrophosphenes. It seems that a more light-sensitive rod system in the retina cannot produce phosphenes at electrical stimulation, while the less light-sensitive cone system can readily react to electric currents. Though paradoxical, the conclusion fits well what is known about the visual appearance of electrophosphenes. Already Purkinje (see in [36]) described the electrophosphene as a mauve color sickle. Dependent on the parameters of stimulations, phosphenes could vary from mauve to yellow, green, or red, but were always colored [36, 107, 108], that is, generated in the cone pathway.
Mechanophosphenes
Deformation phosphenes or mechanophosphenes are light sensations evoked by the deformation of the eyeball. An interesting historical review of mechanophospene studies started from ancient Greece time through Newton and until recently is given in [115]. The results that refute the modern biophoton idea were known from at least the 18th century. It was shown that the deformation phosphenes could be produced at moderate diurnal light intensities. This means that the intensity of the supposed (biophoton) light that generates the phosphenes should be pretty high, at least 7 to 8 orders of magnitude beyond the absolute visual threshold (see above, the section on electrophosphenes). In 1742, Langguth (cited in [115]), in a completely dark room, pressed his eye in front of a mirror, producing phosphenes and trying to see any light emerging from the eye. Nothing was seen. The experiment was repeated with a second observer carefully looking at the stimulated eye of the author. Again, no light leaving the stimulated eye was detected.
Nevertheless, mechanosensitive retinal cells responsible for the mechanophosphenes cannot be reliably identified yet, in contrast to the situation with electrophosphenes and magnetophosphenes. While the slightest touch with a needle tip evokes a tremendous spike response in afferent fibers of ampullae of Lorenzini, their sensitivity to a uniform pressure with a focused ultrasound beam is rather low [113]. Thus an obvious candidate, the photoreceptor or bipolar ribbon synapse, is not firmly supported.
CONCLUSION
Hypothesis on possible visual effects of UPE (biophotons) can be divided into three groups. Firstly, the UPE was considered the source of “retinal dark light” identified with the discrete dark noise of photoreceptors. The idea was tested experimentally; it appeared that the UPE from the retina and other parts of the eye does exist, but its intensity is at least two orders of magnitude lower than necessary to explain the photoreceptor dark noise [65, 68].
Secondly, UPE induced by ionizing radiation (X-rays, γ-rays, and high-energy charged particles) was supposed to be the source of phosphenes observed by astronauts during space flight and by tumor patients treated with hard radiation from particle accelerators. X-rays decidedly convert “dark” rhodopsin into its photoactivated form, Meta II, and evoke a standard electroretinogram response. However, high doses of radiation denature rhodopsin. Therefore, the separation of three possible effects, namely chromophore isomerization by intramolecular energy transfer, UPE production, and protein denaturation is not possible based on available data.
Radiation phosphenes from high-energy particles are partly produced by Cerenkov radiation within the eye. Far larger non-Cerenkov effects causing substantial rhodopsin loss are hypothetically attributed to UPE. However, reported spectroscopically measurable rhodopsin bleaching needs high intensity of visible light, over 8 to 10 orders of magnitude brighter than the UPE from any part of the eye measured till now. No real experimental demonstration of marked rhodopsin bleaching by charged particles via a light-initiated pathway has ever been presented.
Thirdly, the idea of production of electro-, magneto- and deformation phosphenes by biophotons can be discarded based on experimental observations from the 18th–19th centuries. All phosphenes of these sorts do not need dark adaptation and are visible at diurnal illuminance levels. Therefore, the intra-eye light emission supposedly responsible for them can hardly qualify for UPE. Besides, this level of emission would make stimulated eyes shining in the dark which has never been seen by careful observers during the last 200 years.
Список литературы
Gurwitsch A.G. Die Natur des spezifischen Erregers der Zellteilung. Arch. Mikrosk. Anat. und Entw. Mech. 100: 11–40. 1923.
Gurwitsch A.G. Physicalisches über mitogenetische Strahlen. Arch. Mikrosk. Anat. und Entw. Mech. 103: 490–498. 1924.
Gurwitsch L., Anikin A. Das Cornealepithel as Detector and Sender mitogenetischer Strahlung. Wilhelm Roux Arch. Entwichl. Mech. Org. 113: 731–739. 1928.
Volodyaev I., Beloussov LV. Revisiting the mitogenetic effect of ultra-weak photon emission. Front. Physiol. 6: 241. 2015.
Langmuir I. On pathological science. Lecture of 1953 transcribed by R.N. Hall and published in Physics Today. 42: 36–48. 1989.
Sun Y., Wang Ch., Dai J. Biophotons as neural communication signals demonstrated by in situ biophoton autography. Photochem. Photobiol. Sci. 9: 315–322. 2010.
Prasad A., Pospìšil P. Ultraweak photon emission induced by visible light and ultraviolet A radiation via photoactivated skin chromophores: in vivo charge coupled device imaging. J. Biomed. Optics.17: 085004. 2012.
Cifra M., Pospìšil P. Ultra-weak photon emission from biological samples: Definition, mechanisms, properties, detection and applications. J. Photochem. Photobiol. B: Biology. 139: 2–10. 2014.
Ortega-Ojeda F., Calcerrada M., Ferrero A., Campos J., Garcia-Ruiz C. Measuring the human ultra-weak photon emission distribution using an election-multiplying, charge-coupled device as a sensor. Sensors. 18: 1152. 2018.
Novo E., Parola M. Redox mechanisms in hepatic chronic wound healing and fibrogenesis. Fibrogenesis & Tissue Repair. 1: 5. 2008.
Murphy M.E., Sies H. Visible-range low-level chemiluminescence in biological systems. Methods in Enzymology. 186: 595–610. 1990.
Albertin R., Abuja P.M. Monitoring low-density lipoprotein oxidation by low-level chemiluminescence. Free Rad. Res. 29: 75–83. 1998.
Turrens J.F. Mitochondrial formation of reactive oxygen species. J. Physiol. 552 (Pt 2): 335–344. 2003.
Dickinson B.C, Chang C.J. Chemistry and biology of reactive oxygen species in signaling or stress responses. Nature Chem. Biol. 7: 504–511. 2011.
Fedorova G.F., Trofimov A.V., Vasiil’ev R.F., Veprintsev T.L. Peroxy-radical mediated chemiluminescence: mechanistic diversity and fundamentals for antioxidant assay. ARCIVOC. VIII. 163–215. 2007.
Catala´ A. An overview of lipid peroxidation with emphasis in outer segments of photoreceptors and the chemiluminescence assay. Rev. Internat. J. Biochem. & Cell Biol. 38: 1482–1495. 2006.
Fliesler S.J., Anderson R.E. Chemistry and metabolism of lipids in the vertebrate retina. Progress in Lipid Res. 22: 79–131. 1983.
Daemen F.J.M. Vertebrate rod outer segment membranes. Biochim. Biophys. Acta. 300: 255–288. 1973.
Bokkon I., Vimal R.L.P. Retinal phosphenes and discrete dark noises in rods: a new biophysical framework. J. Photochem. Photobiol. B: Biol. 96: 255–259. 2009.
Salari V., Scholkmann F., Bokkon I., Shahbazi F., Tuszynski J. The Physical Mechanism for Retinal Discrete Dark Noise: Thermal Activation or Cellular Ultraweak Photon Emission? PLoS One. 11(3): e0148336. 2016.
Salari V., Scholkmann F., Vimal R.L.P., Csaszar N., Mehdi Aslani M., Bokkon I. Phosphenes, retinal discrete dark noise, negative afterimages and retinogeniculate projections: A new explanatory framework based on endogenous ocular luminescence. Progr. Retinal and Eye Res. 60:101e1. 2017.
Burgos R.C.R., Schoeman J.C., Winden L.J.V., Červinková K., Ramautar R., Van Wijk E.P.A., Cifra M., Berger R., Hankemeier T., Greef J.V. Ultra-weak photon emission as a dynamic tool for monitoring oxidative stress metabolism. Scient. Rep. 7: 1229. 2017.
Gurwitsch A.G., Gurwitsch L.D. Mitogenetic Radiation. M.: Medgiz, 1945. (In Russ).
Ives J.A., van Wijk E.P.A., Bat N., Crawford C., Jonas W.A., Jonas W.B., van Wijk R., van der Greef J. Ultraweak Photon Emission as a Non-Invasive Health Assessment: A Systematic Review. PloS One. 9(2): e87401. 2014.
Murugana N.J., Rouleaub N., Karbowskic L.M., Persinger M.A. Biophotonic markers of malignancy: Discriminating cancers using wavelength-specific biophotons. Biochem. Biophys. Rep. 13: 7–11. 2018.
Calcerrada M., Garcia-Ruiz C. Human Ultraweak Photon Emission: Key Analytical Aspects, Results and Future Trends – A Review. Crit. Rev. Anal. Chem. 49: 368–381. 2019.
Tessaro L.W.E., Dotta B.T., Persinger M.A. Bacterial biophotons as non-local information carriers: Species-specific spectral characteristics of a stress response. Microbiol. Open. 8(6): e00761. 2018.
Mothersill C., Smith R., Wang J., Rusin A., Fernandez-Palomo C., Fazzari J., Seymour C. Biological Entanglement–Like Effect After Communication of Fish Prior to X-Ray Exposure. Dose-Response. Internat. J. Jan. March: 1–17. 2018.
Rahnama M., Tuszynski J.A., Bokkon I., Cifra M., Sardar P., Salari V. Emission of mitochondrial biophotons and their effect on electrical activity of membrane via microtubules. J. Integrat. Neurosci. 10: 65–88. 2011.
Koumar S., Boone K., Tuszynski J.A., Barclay P., Simon K. Possible existence of optical communication channels in the brain. Scient. Rep. 6: 36508. 2016.
Tang R., Dai J. Spatiotemporal imaging of glutamate-induced biophotonic activities and transmission in neural circuits. PloS One. 9: e85643. 2014.
Bókkon I., Salari V., Tuszynski J.A., Antal I. Estimation of the number of biophotons involved in the visual perception of a single-object image: biophoton intensity can be considerably higher inside cells than outside. J. Photochem. Photobiol. B. 100: 160–166. 2010.
Bókkon I. Visual perception and imagery: a new molecular hypothesis. Biosystems. 96: 178–184. 2009.
Cacha L.A., Poznanski R.R. Genomic instantiation of consciousness in neurons through a biophoton field theory. J. Integr. Neurosci. 132: 253–292. 2014.
Wang Z., Wang N., Lib Z., Xiao F., Dai J. Human high intelligence is involved in spectral redshift of biophotonic activities in the brain. PNAS. 113: 8753–8758. 2016.
Helmholtz H. Treatise on physiological optics. Vol. II, English translation. Dover Publications. N.Y. 1962. (Handbuch der physiologischen Optik. 3 Ausgabe. 1909).
Barlow H.B. Retinal noise and absolute threshold. J. Opt. Soc. Am. 46: 634–639. 1956.
de Vries H. Comment to Dr. Wald’s lecture. Documenta Ophthalmol. 3: 137. 1949.
Barlow H.B. Purkinje shift and retinal noise. Nature. 179: 255–256. 1957.
Baylor D.A., Lamb T.D., Yau K.-W. The membrane current of single rod outer segments. J. Physiol. 288: 589–611. 1979.
Baylor D.A., Matthews G., Yau K.-W. Two components of electrical dark noise in toad retinal rod outer segments. J. Physiol. 309: 591–621.1980.
Rieke F., Baylor D.A. Molecular origin of continuous dark noise in rod photoreceptors. Biophys. J. 71: 2553–2572. 1996.
Astakhova L.A., Nikolaeva D.A., Fedotkina T.V., Govardovskii V.I., Firsov M.L. Elevated cAMP improves signal-to-noise ratio in amphibian rod photoreceptors. J. Gen. Physiol. 149: 689–701. 2017.
Lamb T.D., Heck M., Kraft T.W. Implications of dimeric activation of PDE6 for rod phototransduction. Open Biol. 8: 180076. 2018.
Baylor D.A., Lamb T.D., Yau K.-W. Responses of retinal rods to single photons. J. Physiol. 288. 613–634. 1979.
Lamb T.D., Pugh E.N. Jr. Phototransdution, dark adaptation, and rhodopsin regeneration. The Proctor lecture. Investigat. Ophthalmol. Visual Sci. 47: 5138–5152. 2006.
Arshavsky V.Y., Burns M.E. Photoreceptor signaling: supporting vision across a wide range of light intensities. J. Biol. Chem. 287: 1620–1626. 2012.
Arshavsky V.Y., Burns M.E. Current understanding of signal amplification in phototransduction. Cell. Logistics. 4: e29390. 2014.
Lamb T.D., Hunt D.M. Evolution of the vertebrate phototransduction cascade activation steps. Dev. Biol. 431: 77–92. 2017.
Lamb T.D., Patel H.R., Chuah A., Hunt D.M. Evolution of the shut-off steps of vertebrate phototransduction. Open Biol. 8: 170232. 2018.
Donner K. Spectral and Thermal Properties of Rhodopsins: Closely Related but not Tightly Coupled. Russ. J. Physiol. 106(4): 421–435. 2020. https://doi.org/10.31857/S086981392004001
Firsov M. L., Govardovskii V.I. Dark noise of visual pigments with different absorption maxima. Sensornye Sistemy. 4: 25–34. 1990. (In Russ).
Donner K., Firsov M.L., Govardovskii V.I. The frequency of isomerization-like”dark” events in rhodopsin and porphyropsin rods of the bull-frog retina. J. Physiol. 428: 673–692. 1990.
Firsov M. L., Donner K., Govardovskii V.I. pH and rate of ‘‘dark’’ events in toad retinal rods: test of a hypothesis on the molecular origin of photoreceptor noise. J. Physiol. 539(3): 837–846. 2002.
Luk H.L., Bhattacharyya N., Montisci F., Morrow J.M., Melaccio F., Wada A., Sheves M., Fanelli F., Chang B.S.W., Olivucci M. Modulation of thermal noise and spectral sensitivity in Lake Baikal cottoid fish rhodopsins. Scient. Rep. 6: 38425. 2016.
Luo D.G., Yue W.W., Ala-Laurila P., Yau K.-W. Activation of visual pigments by light and heat. Science. 332: 1307–1312. 2011.
Gozem S., Schapiro I., Ferré N., Olivucci M. On the molecular mechanism of thermal noise in rod photoreceptors. Science. 337: 1225–1228. 2012.
Yanagawa M., Kojima K., Yamashita T., Imamoto Y., Matsuyama T., Nakanishi T., Yamano Y., Wada A., Sako Y., Shichida Y. Origin of the low thermal isomerization rate of rhodopsin chromophore. Scient. Rep. 5: 11081. 2015.
Barlow R.B. Jr., Birge R.R., Kaplan E., Tallent J.R. On the molecular origin of photoreceptor noise. Nature. 366: 64–66. 1993.
Barlow R.R., Birge R.B. On the molecular origins of thermal noise in vertebrate and invertebrate photoreceptors. Biophys. Chem. 55: 115–126. 1995.
Sampath A.P., Baylor D.A. Molecular Mechanism of Spontaneous Pigment Activation in Retinal Cones. Biophys. J. 83: 184–193. 2002.
Fu Y., Kefalov V., Luo D.-G., Xue T., Yau K.-W. Quantal noise from human red cone pigment. Nat. Neurosci. 11: 565–571. 2008.
Bókkon I., Vimal R.L.P. Retinal phosphenes and discrete dark noises in rods: A new biophysical framework. J. Photochem. Photobiol. B. 96: 255–259. 2009.
Wang C., Bókkon I., Dai J., Antal I. Spontaneous and visible light-induced ultraweak photon emission from rat eyes. Brain Res. 1369: 1–9. 2011.
Li Z., Dai J. Biophotons Contribute to Retinal Dark Noise. Neurosci. Bull. 32: 246–252. 2016.
Salari V., Scholkmann F., Bokkon I., Shahbazi F., Tuszynski J. The physical mechanism for retinal discrete dark noise: Thermal activation or cellular ultraweak photon emission? PLoS One. 11: e0148336. 2016.
Salari V., Scholkmann F., Vimal R.L.P., Császár N., Aslani M., Bókkon I. Phosphenes, retinal discrete dark noise, negative afterimages and retinogeniculate projections: A new explanatory framework based on endogenous ocular luminescence. Prog. Retin. Eye Res. 60: 101–119. 2017.
Govardovskii V.I., Astakhova L.A., Rotov A.Yu., Firsov M.L. Rejection of the biophoton hypothesis on the origin of photoreceptor dark noise. J. Gen. Physiol. 151: 887–897. 2019.
Craik K.J.W. Origin of visual after-images. Nature. 145: 512. 1940.
van Lier R., Vergeer M., Anstis S. Filling-in afterimage colors between the lines. Curr. Biol. 19: R323–R324. 2009.
Powell G., Bompas A., Sumner P. Making the incredible credible: Afterimages are modulated by contextual edges more than real stimuli. J. Vision. 12: 1–13. 2012.
Zeki S., Cheadle S., Pepper J., Mylonas D. The constancy of colored after-images. Front. Hum. Neurosci. 11: 229. 2017.
Rotov A.Yu., Astakhova L.A., Firsov M.L., Govardovskii V.I. Light Adaptation of Retinal Rods, Adaptation Memory and Afterimages. Russ. J. Physiol. 106: 462–473. 2020. (In Russ).
Govardovskii V.I., Fyhrquist N., Reuter T., Kuzmin D., Donner K. In search of the visual pigment template. Visual Neurosci. 17: 509–528. 2000.
Griffin D.R., Hubbard R., Wald G. The sensitivity of the human eye to infra-red radiation. J. Opt. Soc. Am. 37: 546–554. 1947.
Pinegin N.I. Scotopic (rod) sensitivity of human eye in red and infrared spectrum. Proc. Acad. Sci. USSR. 56: 811–814. 1947. (In Russ.)
Govardovskii V.I., Zueva L.V. Spectral sensitivity of the frog eye in the ultraviolet and visible region. Vision Res. 14: 1317–1321. 1974.
Kropf A. Intramolecular energy transfer in rhodopsin. Vision Res. 7: 811–818. 1967.
Lipetz L.E. The X-ray and radium phosphenes. Br. J. Ophthalmol. 39: 577–598. 1955.
Lipetz L.E. Electrophysiology of the x-ray phosphene. Radiat. Res. 2: 306–329. 1955.
Steidley K.D. The Radiation Phosphene. Vision Res. 30: 1139–1143. 1990.
Bachofer C.S., Wittry S.E. Electroretinogram in response to x-ray stimulation. Science. 133: 642–644. 1961.
Doly M., Isabelle D.B., Vincent P., Gaillard G., Meyniel G. Mechanism of the formation of X-ray-induced phosphenes. I. Electrophysiological investigations. Radiat. Res. 82: 93–105. 1980.
Peskin J.C. The effects of irradiation upon visual purple. Am. J. Ophthalmol. 39: 849–854. 1955.
Doly M., Isabelle D.B., Vincent P., Gaillard G., Meyniel G. Mechanism of the formation of X ray-induced phosphenes. II. Photochemical investigations. Radiat. Res. 82: 430–440. 1980.
Pinsky L.S., Osborne W.Z., Bailey J.V., Benson R.E., Thompson L.F. Light flashes observed by astronauts on Apollo 11 through Apollo 17. Science. 183: 957–959. 1974.
Pinsky L.S., Osborne W.Z., Hoffman R.A., Bailey J.V. Light flashes observed by astronauts on Skylab 4. Science. 188: 928–930. 1975.
Budinger T.F., Tobias C.A., Huesman R.H., Upham F.T., Wieskamp T.F., Hoffman R.A. Apollo–Soyuz light-flashes observations. Life Sci. Space Res. 15: 141–146. 1977.
Bidoli V., Casolino M., De Pascale M.P., Furano G., Morselli A., Picozza L.P., Reali E., Sparvoli R., Galper A.M., Ozerov A.V., Popov Yu.V., Vavilov N.R., Alexandrov A.P., Avdeev S.V., Barbiellini G., Bonvicini W., Vacchi A., Zampa N., Bartalucci S., Mazzenga G., Ricci M., Adriani O., Spillantini P., Boezio M., Carlson P., Fuglesang C., Castellini G., Sannita W.G. Study of cosmic rays and light flashes on board space station MIR: the SilEye experiment. Adv. Space Res. 25: 2075–2079. 2000.
Fuglesang C., Narici L., Picozza P., Sannita W.G. Light flashes in low Earth orbit: survey responses from 59 astronauts. Aviat. Space Envir. Med. 77: 449–452. 2006.
Sannita W.G., Narici L., Picozza P. Positive visual phenomena in space: a scientific case and a safety issue in space travel. Vision Res. 46: 2159–2165. 2006.
Schardt D., Kavatsyuk O., Krämer M., Durante M. Light flashes in cancer patients treated with heavy ions. Brain Stimul. 6: 416–417.2013.
Mathis T., Vignot S., Leal C., Caujolle J.-P., Maschi C., Mauget-Faÿsse M., Kodjikian L., Baillif S., Herault J., Thariat J. Mechanisms of phosphenes in irradiated patients. Oncotarget. 8: 64579–64590. 2017.
McNulty P.J., Pease V.P., Pinsky L.S., Bond V.P., Schimmerling W., Vosburgh K.G. Visual sensations induced by relativistic nitrogen nuclei. Science. 178: 160–162. 1972.
McNulty P.J., Pease V.P., Bond V.P. Comparison of the light-flash phenomena observed in space and in laboratory experiments. Life Sci. Space Res. 15: 135–140. 1977.
McNulty P.J., Pease V.P., Bond V.P. Visual sensations induced by relativistic pions. Radiat. Res. 66: 519–530. 1976
McNulty P.J., Pease V.P., Bond V.P. Muon-induced visual sensations. J. Opt. Soc. Am. 66: 49–55. 1976.
McNulty P.J., Pease V.P., Bond V.P. Visual Phenomena Induced by Relativistic Carbon Ions With and Without Cerenkov Radiation. Science. 201: 341–343. 1978.
Trukhanov K.A., Brindikova T.A., Zak P.P., Lebedev B.M., Spassky A.V., Fedorovich I.B., Ostrovsky M.A. Effects of charged heavy particles on rhodopsin and isolated retina of the eye. Proceed. Russ. Acad. Sci. 377: 715–717. 2001. (In Russ).
Narici L., Paci M., Brunetti V., Rinaldi A., Sannita W.G., De Martino A. Bovine rod rhodopsin. 1. Bleaching by luminescence in vitro by recombination of radicals from polyunsaturated fatty acids. Free Radical Biol. Med. 53: 482–487. 2012.
Narici L., Paci M., Brunetti V., Rinaldi A., Sannita W.G., Carozzo S., De Martino A. Bovine rod rhodopsin: 2. Bleaching in vitro upon 12C ions irradiation as source of effects as light flash for patients and for humans in space. Internat. J. Radiat. Biol., Early Online: 1–5. 2013.
Boll F. Zur Anatomie und Physiologie der retina. Arch. Anat. Physiol .(Physiol Abt). 1877: 4–36. (Translated into English and reprinted: Boll F. On the anatomy and physiology of the retina.) Vision Res. 17: 1249–1265. 1977.
DeGrip W.J., Rothschild K.J. Structure and mechanisms of vertebrate visual pigments. In Handbook of Biological Physics. Vol. 3. Eds. Stavenga D.G., DeGrip W.J. & Pugh E.N. Jr. Chapt. 1: 1–54. Elsevier Science B.V. North Holland. 2000.
Lewis J.W., van Kuijk F.J.G.M., Carruthers J.A., Kliger D.S. Metarhodopsin III formation and decay kinetics: Comparison of human and bovine rhodopsin. Vision Res. 37: 1–8. 1997.
Korenyak D.A., Govardovskii V.I. The effect of temperature on the slow stages of photolysis of rhodopsin in intact rods of frog and rat retinas. Sensory Systems. 26: 141–149. 2012. (In Russ).
Brindley G.S. The site of electrical excitation of the human eye. J. Physiol. 127: 189–200. 1955.
Carpenter R.H. Electrical stimulation of the human eye in different adaptational states. J. Physiol. 221: 137–148. 1972.
Wolff J.G., Delacour J., Carpenter R.H.S., Brindley G.S. The patterns seen when alternating electric current is passed through the eye, Quart. J. Exp. Psychol. 20: 1–10. 1968.
Lövsund P., Ōberg P.Å., Nilsson S.E.G., Reuter T. Magnetophosphenes: a quantitative analysis of thresholds. Med. & Biol. Eng. & Comput. 18: 326–334. 1980.
Attwell D. Interaction of low frequency electric fields with the nervous system: the retina as a model system. Radiat. Prot. Dosimetry. 106: 341–348. 2003.
Matthews G., Fuchs P. The diverse roles of ribbon synapses in sensory neurotransmission. Nat. Rev. Neurosci. 11(12): 812–22. 2010.
Kalmijn A.J. Electric and magnetic field detection in elasmobranch fishes. Science. 218: 916–918. 1982.
Brown G.R., Ilyinsky O.B. Physiology of electroreceptors. Nauka Publ. Leningrad. 1984. (In Russ).
Schneeweis D.M., Schnapf J.L. Photovoltage of rods and cones in the macaque retina. Science. 268: 1053–1056. 1995.
Grüsser O.-J., Hagner M. On the history of deformation phosphenes and the idea of internal light generated in the eye for the purpose of vision. Documenta Ophthalmol. 74: 57–85. 1990.
Дополнительные материалы отсутствуют.
Инструменты
Российский физиологический журнал им. И.М. Сеченова