Российский физиологический журнал им. И.М. Сеченова, 2020, T. 106, № 4, стр. 421-435
Spectral and Thermal Properties of Rhodopsins: Closely Related but not Tightly Coupled
K. Donner *
Molecular and Integrative Biosciences Research Program Faculty of Biological and Environmental Sciences University of Helsinki
Helsinki, Finland
* E-mail: kristian.donner@helsinki.fi
Поступила в редакцию 8.02.2020
После доработки 21.02.2020
Принята к публикации 21.02.2020
Аннотация
Rhodopsins, the primary molecules of vision in all seeing animals, can be activated not only by photon energy (light) but also by thermal energy (heat). Spectral absorbance is evolutionarily tuned by critical residues in the amino acid sequence of the protein part (opsin), which affect the energy needed for 11-cis → all-trans isomerization of the covalently bound chromophore. Already in the 1940’s it was suggested that high sensitivity to long-wavelength light, being indicative of a low energy barrier for activation, should correlate with high probability for thermal activation, and that randomly occurring thermal activations would constitute an irreducible noise setting absolute constraints for the detection of weak light signals. This idea has received strong experimental as well as theoretical support over the last 40 years. Most of the experimental evidence comes from physiological studies of light responses and dark noise in the light-sensitive current of vertebrate photoreceptor cells. Here I review this work, which has firmly established the correlation of spectral sensitivity and thermal noise and led to new theoretical insights. On the other hand, there remains significant freedom for independent adjustment of the two variables by tinkering with the opsin. This is a question of fundamental evolutionary as well as practical interest.
All seeing animals use basically the same molecule for capturing photons and triggering the phototransduction cascade. The molecule, generically referred to as (visual, or “type 2”) rhodopsin, consists of a 7-transmembrane G-protein-coupled receptor, opsin, to which a light-sensitive prosthetic group, the chromophore, is covalently bound [1, 2]. The chromophore is always some form of retinaldehyde (retinal); in vertebrates derived either from vitamin A1 (11-cis retinal, A1) or A2 (11-cis 3,4-didehydroretinal, A2). Use of the latter is almost entirely restricted to fishes and amphibians [3] (but see [4]), requiring that the animal possess the enzyme Cyp27c1 necessary for synthesizing A2 from A1 [5]. The most important functional variable of visual pigments is the absorbance spectrum, expressing the probability of absorbing photons as function of their energy, usually displayed as the fraction of light absorbed as function of wavelength. Absorbance spectra can be uniquely defined by a template (one for A1 and one for A2 pigments) with a single variable, the wavelength of maximum absorbance (λmax), which defines both the spectral position and the width of the spectrum [6]. Switching from A1 to A2 chromophore in the same opsin may be used for red-shifting λmax on a physiological time scale [7, 8], while the universal way of tuning the absorbance spectrum of functional visual pigments is by mutations in the amino-acid sequence of the opsin, effective on evolutionary time scales.
It is a priori reasonable to think that a molecule designed to be activated by absorbing light energy cannot be perfectly stable against activation by thermal energy. H. Autrum [9] first pointed out that random activation of rhodopsin molecules would constitute a light-identical shot noise setting an ultimate limit to the detection of real photons, and H.B. Barlow [10] showed that the statistics of human light detection near the absolute threshold is consistent with this idea. E.J. Denton and M.H. Pirenne [11] had previously estimated an upper limit for the possible rate of occurrence of such activations in humans, translating into <0.3 rod–1 s–1 in a rod with ~108 rhodopsin molecules, while emphasizing that the rate could in fact be so low as to lack any significance. Whatever the exact value, it was clear that such rare events could not be approached by conventional biochemical methods.
DISCRETE “DARK” EVENTS IN RODS AS PROXIES FOR THERMAL ACTIVATION OF RHODOPSIN
In the late 1970’s, it became possible to “see” single-rhodopsin events electrophysiologically, taking advantage of the powerful molecular amplification of phototransduction in dark-adapted rod cells. The suction-electrode technique developed by K.W. Yau et al. [12], inspired by E. Neher and B. Sakmann [13], allowed recording the light-sensitive current of single rods essentially free from confounding effects of rod-rod coupling and voltage-sensitive channels. The first recordings were from the sturdy rods of the cane toad, Bufo marinus11. Under very dim background illumination, discrete current bumps of fairly standardized shape and size could be discerned, Poisson-distributed in time, as expected from random arrival and absorption of photons [14]. The size of these quantal responses (SQRs) was ~1 pA at peak, representing a ~5% decrease of the circulating current in a dark-adapted rod. During the following decades, the molecular events that shape most aspects of rod and cone responses to light have been clarified in considerable detail through a fruitful interaction of biochemical and electrophysiological studies. Precisely how the fairly reproducible SQRs are generated has been one of the most challenging questions. Full consensus has not yet been reached, but the crucial variables of the amplification [15–18] and termination reactions are now known with reasonable quantitative precision [19–25].
D.A. Baylor et al. [26] found that even in absolute darkness there still occurred occasional discrete current bumps indistinguishable from the SQR. The obvious hypothesis was that these “dark events” originate at the same point as the SQR, at the very input to the amplification cascade, i.e., in the rhodopsin molecule, rather than arising e.g. from bursts of fortuitous synchronous activation of large numbers of intermediates in the cascade (G-proteins or PDE molecules). Thus they seemed to offer an exceptional window into the “dark life” of the rhodopsin molecule.
This notion faced a serious problem, though. From the temperature-dependence of the rates of dark events, D.A. Baylor and colleagues had determined an activation energy of ~22 kcal mol–1 [26]. They stated that this value “seem(s) consistent with isomerization of the 11-cis retinal chromophore as the mechanism for thermal activation”, because it was close to that determined for thermal isomerization of the chromophore in aquaeous digitonin (24.5 kcal mol–1) [27]. About the same time, however, A. Cooper [28] showed that the ground-state energy of the early photobleaching product, bathorhodopsin, is 35 kcal mol-1 higher than that of rhodopsin, and argued for a 45 kcal mol–1 energy barrier for the ground-state (thermal) 11-cis → all-trans transition. Neither did D.A. Baylor et al. cite two earlier studies of frog rhodopsins in solution: R.J. Lythgoe and J.P. Quilliam [29] had estimated an activation energy of 44 kcal mol–1 for thermal bleaching, and R.C.C. St. George [30] had arrived at a photoactivation energy of 48.5 kcal mol–1 based on the longest wavelength (590 nm) where photon energy alone sufficed for activation (see below). I shall hereafter denote by Ea and EaH the energies for activation by light and by heat, respectively – estimates as well as the underlying entities, even though this may occasionally cause some confusion.
The discrepancy between apparent activation energies for photic and thermal activation caused much speculation on differing molecular routes, stressing that there is actually no reason why Ea and EaH should be the same. R.B. Barlow and colleagues [31] first came up with a testable hypothesis. They proposed that thermal events originate in a small (<0.01%) subpopulation of rhodopsin molecules where the Schiff-base linkage between chromophore and opsin is unprotonated. According to their molecular modelling, this could lower the activation energy for ground-state 11-cis → all-trans isomerization by about half. Experimental testing is straightforward in principle, since the proportion of rhodopsin molecules with unprotonated Schiff base must increase with alkalinization and decrease with acidification in predictable manner. In Limulus, they found that correlated decreases in pH and in the activity of optic nerve fibers could be induced by efferent stimulation. However, the metric they used, spiking in the afferent nerve, is at least twice removed from the rhodopsin molecule. First, decreased activity may result from some factor other than a decreased rate of thermal “quantum bumps” in the photoreceptors (e.g. acidification as such). Second, “quantum bumps” in Limulus, originally reported by S. Yeandle [32], do not bear a straightforward relation to activation of single rhodopsin molecules [33]. Subsequent experiments on toad rods [34] and salamander cones [35] indicated no relevant effect of changing pH (intra- and extracellular) on thermal event rates. The deprotonation hypothesis could be rejected.
A second hypothesis disrupting the connection between SQR-like “dark” events in rods and intrinsic properties of the rhodopsin molecule has been advanced by I. Bókkon and R.L.P. Vimal [36]. They proposed that the events are in fact responses to real photons, “biophotons”, emitted by the retinal tissue. However, V.I. Govardovskii and coworkers [37] showed by direct measurements that biophoton emission rates in frog and sterlet retina are >100-fold too low to account for the discrete rod events recorded in the same species.
A third possibility that could cast doubt on the use of light-like noise as a measure of thermal rhodopsin activation is if cannot, after all, be distinguished from noise triggered at a later stage of the phototransduction cascade. A recent study [18] shows that the first amplification step, the number of G-proteins activated per activated rhodopsin, in dark-adapted mouse rods is only 12–14 rather than the commonly quoted number ~100, and that PDE-initiated events may be more similar to the SQR than previously thought. This could explain the difficulty of separating SQR-like events from “continuous noise” in mouse rods [38], but quantitative relations are likely to vary between species. In many species SQRs are much more distinct from continuous noise [39].
ESTIMATES OF THERMAL AND PHOTIC ACTIVATION ENERGY RECONCILED
The problem of the 2-fold discrepancy between estimates of photic [28, 40, 41] and thermal [26] activation energies remained unresolved for more than 20 years. The Gordian knot was cut by P. Ala-Laurila and collegues [42] who argued that the low thermal estimate was no more than an analytical artifact. R.J. Lythgoe and J.P. Quilliam [29] had already in 1938 considered whether rhodopsin activation kinetics would be affected by complexities dealt with in a recent treatise by C.N. Hinshelwood [43], and R.C.C. St. George [30] and P.R. Lewis [44] had applied some aspects of it, but only in [42] were its full implications developed. Briefly, the thermal energy distribution of complex molecules like rhodopsin, or even the 11-cis retinaldehyde chromophore, cannot be described by simple Boltzmann statistics, but must take into account the internal energy of the molecule present in a large number of vibrational modes. The chromophore alone consists of n = 49 atoms and has 3n – 6 = 141 kinetic degrees of freedom. The number of vibrational modes n (≤141) that actually contribute towards 11-cis → all-trans isomerization in a given opsin environment is unknown and could depend e.g. on the amino acid residues around the chromophore pocket. The predicted effect of temperature on the fraction F of molecules exceeding EaH, and hence on dark event rates, will depend strongly on n, implying that the temperature-dependence of D.A. Baylor et al’s [26] data shown as an Arrhenius plot in Fig. 2 will yield very different values of EaH depending on what n value is assumed. Based on Boltzmann statistics, the slope of the straight line indeed gives EaH = 21.9 kcal mol–1, but based on Hinshelwood statistics it may give, for example, EaH = Ea = 44.3 kcal mol–1 (where Ea is the estimated photoactivation energy of Bufo marinus rhodopsin in [45]) if n = 79, or EaH = = 34.3 kcal mol–1 if n = 44. The last example was given by P. Ala-Laurila et al. [42] to show the robustness of their model against a possible 5–10 kcal mol–1 difference between the electronically excited state and the peak of the ground-state energy barrier (Ea – EaH) as suggested by molecular modelling [46, 47]. Obviously, their approach did not enable actual estimation of EaH, but it removed the supposed incompatibility with estimates of Ea, allowing that thermal activation may follow the same molecular pathway as photoactivation, starting from 11-cis → all-trans isomerization of the chromophore.
This is consistent with current molecular understanding. The energies of the ground-state barrier for thermal activation and the electronically excited state induced by photon absorption are expected to be close in view of the femtosecond transition from the latter to the earliest identified ground-state photoproduct [46–48]. Quantum chemical modelling [49] suggests that the transition state mediating thermal activation has the same electronic structure as the excited state, manifesting intrinsic chromophore features associated with the existence of a conical intersection between the ground and excited states (cf. [50]). Importantly, this gives a theoretical, molecular-level foundation for a correlation between the wavelength of maximum absorbance λmax and the rate of dark events k (“the Barlow correlation”). The model has recently been applied to the opsins of the endemic cottoid fishes of Lake Baikal in an attempt to identify specific amino acid residues that may regulate both spectral and thermal properties in connection with the blue-shift of corresponding pigments between species with increasing habitat depth [51].
EMPIRICAL TESTING OF “BARLOW’S HYPOTHESIS”
The basically simple idea that long-wavelength sensitivity should correlate with high thermal activation rates first appears in the literature in a brief comment by H. de Vries in 1949 [52]: high sensitivity to long light wavelengths (low-energy photons) entails a low energy barrier for activation, and this will imply a high probability that the barrier be surmounted by molecular thermal energy alone. The conceptual relations between activation energy Ea, spectral absorbance (captured by λmax) and the fraction F of rhodopsins with thermal energy exceeding Ea are shown by the scheme in Fig. 1. H.B. Barlow [53] gave the idea its classical formulation, proposing it as a teleological explanation for the ubiquitous blue-shift of night vision compared with daylight vision (the Purkinje shift). He pointed out that the shift does not increase photon catch at night, since star- and moonlight is in fact somewhat more “reddish” than daylight, but could be useful as a means of decreasing thermal noise.
Fig. 1.
General scheme of the presumed interrelations (arrows marked ①, ② and ③) between three main functional variables of rhodopsins. Values for all of these can be derived from electrophysiological experiments on the light-sensitive current of photoreceptor cells. Both the absorption spectrum and the thermal activation rate are functions of the activation energy Ea, hypothesized to be the same or close and well-correlated for photic and thermal activation. As a consequence, the absorption spectrum and the thermal event rate are correlated (dashed arrow ③). Barlow (1957) [53] initially assumed ① λmax = hc/Ea, ② F = exp(–Ea /kT) according to Boltzmann statistics, but envisaged that the assumptions could be improved, referring to Lewis (1955) [44], who replaced λmax in ① by a wavelength λ0 > λmax and suggested the use of Hinshelwood statistics. A new model based on these ideas was fully developed by Ala-Laurila et al. (2004) [42], who based ①on the regression of Ea on λmax in an empirical data set, and ② on the fraction of molecules with thermal energy ≥ Ea according to Hinshelwood statistics (see Text).
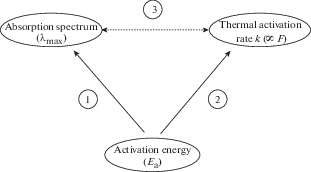
Fig. 2.
Arrhenius plot of the temperature-dependence of rates of dark SQR-like events in rods of Bufo marinus Each symbol type marks data from one rod in Table 2 of Baylor et al (1980) [26]. The least-square regression line fitted to the points gives different estimates EaH for the thermal activation energy depending on the underlying assumptions: (i) conventional Arrhenius analysis relying on Boltzmann statistics for simple particles as in [26] gives EaH = 21.9 kcal/mol; (ii) Hinshelwood statistics for complex molecules assuming molecular vibration modes n = 79, gives thermal activation energy EaH = 44.3 kcal/mol, equal to the photoactivation energy Ea estimated from rods of the same species in [45]; (iii) Hinshelwood statistics assuming n = 44 gives EaH = 34.3 kcal mol–1, which would accommodate a possible energy gap of 5–10 kcal mol–1 between Ea and EaH [42].
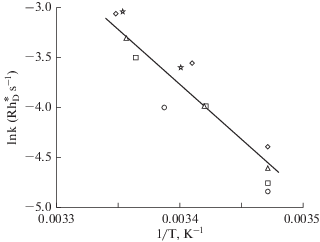
H.B. Barlow’s hypothesis inspired several experimental studies of dark noise in rods with different spectral sensitivities even while the conflict between the estimated values EaH and Ea remained unresolved. The first of these, on the blue-sensitive (433-nm) “green” rods of toad, provided a disappointment, as estimated dark event rates per pigment molecule were more than 4 times higher than in the regular 503-nm rods ([54] c.f. however [39] and below). We now know that the pigment of these blue-sensitive rods is not a rod rhodopsin (Rh1), but a cone (SWS2) pigment, albeit with a stabilizing mutation [55, 56]. Luckily, studies on bullfrog rods with A1 (502 nm) and A2 (525 nm) pigment [57] and sturgeon (A2) rods with λmax = 538 and 549 nm [58] were more encouraging, showing a clear correlation between long-wavelength sensitivity and high rates of SQR-like dark events. This kept interest in Barlow’s hypothesis alive.
THE PHOTOACTIVATION ENERGY Ea
In the general scheme (Fig. 1), the activation energy Ea is in a pivotal position, being the determinant of both spectral absorbance and rates of thermal events. H.B. Barlow [53] initially assumed that Ea would be equal to the photon energy at the wavelength of maximum absorption or “maximum visibility” (Ea = hc/λmax), but he envisaged improvements by taking into account, inter alia, “Lewis’s further development of Stiles’s theory”. One such development was the realization that Ea corresponds to the photon energy not at λmax, but at some wavelength λ0 > λmax, recognizable as the longest wavelength where activation can still occur without supplementation by thermal energy [30, 44, 59]:
(1)
${{E}_{a}} = {{hc} \mathord{\left/ {\vphantom {{hc} {{{\lambda }_{0}}}}} \right. \kern-0em} {{{\lambda }_{0}}}} = {{{\text{C}}hc} \mathord{\left/ {\vphantom {{{\text{C}}hc} {{{\lambda }_{{{\text{max}}}}}}}} \right. \kern-0em} {{{\lambda }_{{{\text{max}}}}}}},$Summarizing results of their measurements on 12 photoreceptor species (both rods and cones with both A1 and A2 pigments, and two pigments in crustacean rhabdoms), Ala-Laurila et al. (2004) [64] could not confirm the simple relation expressed by eqn. (1), but still found a significant correlation between Ea and 1/λmax described by the following linear regression equation:
(2)
${{E}_{a}} = 7.10{\text{ }}{{{\text{kcal}}} \mathord{\left/ {\vphantom {{{\text{kcal}}} {{\text{mol }}}}} \right. \kern-0em} {{\text{mol }}}} + 19\,800{\text{ nm }}{{{\text{kcal}}} \mathord{\left/ {\vphantom {{{\text{kcal}}} {{\text{mol}}}}} \right. \kern-0em} {{\text{mol}}}} \times \left( {{1 \mathord{\left/ {\vphantom {1 {{{\lambda }_{{{\text{max}}}}}}}} \right. \kern-0em} {{{\lambda }_{{{\text{max}}}}}}}} \right)\,\,{\text{n}}{{{\text{m}}}^{{ - 1}}}.$The coefficient of determination was only 0.73, however, implying that 27% of the variance remained unexplained variation around the regression line. Moreover, the line itself is less steep than expected.
The data underlying eqn. (2) had been obtained by a combination of microspectrophotometry and transretinal ERG recording potentially susceptible to several sources of error, which moreover may differ between species. D.G. Luo et al. [39] reexamined the Ea – 1/λmax relation in 7 species of vertebrate rods and cones, recording spectral sensitivities with the more precise suction-pipette technique. They did find a close agreement with eqn. (1), with λ0 as a remarkably constant multiple of λmax across species (mean ratio λmax/λ0 ± SD = 0.84 ± 0.01). Strict comparison between [64] and [39] is largely impossible, though, as they are based on mainly non-overlapping samples of photoreceptor species. For the Bufo marinus “red” rod included in both, the reported values differ significantly (Ea = 44.3 kcal mol–1, λmax = 503 nm, λmax/λ0 = 0.78 in [64] versus Ea = 48.0 kcal mol–1, λmax = 500 nm, λmax/λ0 = 0.84 in [39]).
While there is no doubt of the superior quality of the suction-pipette recordings in [39], the differences cannot be lightly dismissed as being due to poor quality of the ERG data. The presence of true variation in λmax/λ0 between species is suggested by a comparison of the Ea values of two A1–A2 pigment pairs (Fig. 3 based on ERG, cf. Fig. 3A in [64]). One pair consists of L-cone pigments of juvenile (A2 with λmax = 629 nm) and adult (A1 with λmax = 562 nm) Rana temporaria (original data from [61]), the other pair of rod pigments from adult Rana catesbeiana (A2 pigment with λmax = 525 nm and A1 pigment with λmax = = 502 nm; original data from [62]). The two straight lines plot eqn. (1) with λmax/λ0 = 0.89 for the R. temporaria L-cones (blue) and 0.81 for the R. catesbeiana rods (green). The good fit of the two different lines to the respective pair of points suggests three things. First, upon a chromophore switch in the same opsin, changes in Ea and λmax may indeed be tightly coupled as described by eqn. (1). Second, random variation of the ERG-based estimates seems to be fairly small, because otherwise one would expect larger variation of λmax/λ0 within each pair. Third, as there were no clear sources of systematic error liable to differentially affect estimates for these two species of frogs, the ca 10% difference in λmax/λ0 between the two pairs appears significant. Pending new data, a cautious conclusion is that the relative shallowness of the regression equation (2) does reflect a real biological trend. One may hypothesize that the evolution of opsins that confer high long-wavelength-sensitivity has also involved selection against a “default” decrease in activation energy, as far as decoupling of the two by tinkering with the amino acid sequence is possible.
Fig. 3.
Photoactivation energies of two A1–A2 pigment pairs. Data points marked RT represent L-cone pigments of Rana temporaria (λmax = 562 nm (A1) and 629 nm (A2), values from [61]. Data points marked RC represent rod pigments of Rana catesbeiana (λmax = 502 nm (A1) and 525 nm (A2), values from [62, 57 ]. Both lines are described by the general equation Ea = hc/λ0, where λ0 = 1.12 λmax for the RT L-cones (blue line) and 1.23 λmax for the RC rods (green line). Reproduced from [64].
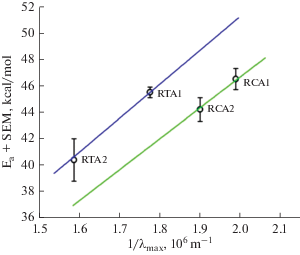
SYNTHESIS: RATES OF THERMAL ACTIVATIONS VS. SPECTRAL ABSORBANCE
From the viewpoint of visual function, what finally matters is the resultant relation between λmax and the rate of randomly occurring thermal activations k (dashed arrow ③ in Fig. 1). P. Ala-Laurila et al. [42] compared the data then available for rods and cones with their model, where k was predicted by using the empirical equation (2) for the Ea – 1/λmax relation, and the fraction of molecules with energy exceeding Ea was obtained from Hinshelwood’s distribution for n = 79 (the same value that made EaH = Ea for Bufo marinus rods in Fig. 2). Fig. 4 plots the comparison as lg k against 1/λmax for rods (A) and cones (B), reproduced from [42]. The solid lines show the model prediction, where the vertical positioning of each line is the only parameter freely fitted. This corresponds to fixing the “pre-exponential factor” in the Arrhenius equation, i.e., fixing the absolute rates of dark events (which are some three orders of magnitude higher in cones than in rods). The slopes of the lines provide an acceptable description of the admittedly sparse and scattered data for both rods and cones, in qualitative agreement with Barlow’s hypothesis. The prediction of Barlow’s original formulation (dashed line) is shown for comparison. The dotted lines show the “robustness test”, i.e. the model prediction assuming a 10 kcal mol–1 difference between Ea and EaH (whereby n = 44 is assumed, based on the fit to the temperature data in Fig. 2).
Fig. 4.
The relation between rates of thermal dark events per rhodopsin molecule k [${\text{R}}_{{\text{D}}}^{*}$ s–1] and spectral sensitivity measured by the wavelength of peak absorbance λmax in rods (A) and cones (B). Rates k were estimated from dark noise recordings in single cells, and spectral absorbance by microspectrophotometry. Note that the plot of –lg k against 1/λmax [106 m–1] places the noisiest and most long-wavelength-sensitive pigments at the bottom left and the most stable and short-wavelength sensitive ones at the upper right. In both panels the full-drawn line represents the model of Ala-Laurila et al. (2004) [42] with the same number of molecular vibration modes (n = 79), i.e., the number that provided the best fit to the Bufo rod temperature data in Fig. 2, combined with the relation between Ea and 1/λmax given by eqn. (2). The dotted lines show the model prediction assuming a 10 kcal/mol Ea – EaH difference and n = 44 (see Text). The dashed lines show the prediction of Barlow’s hypothesis as originally formulated [53]. Reproduced from Figs. 2 and 3 in [42].
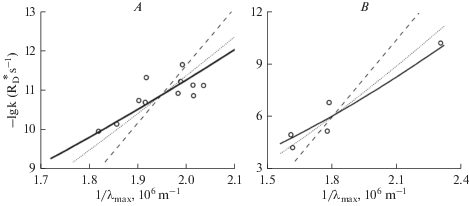
Again, D.G. Luo et al. [39] provided new data of reference quality against which the earlier results must be assessed. They mainly found a much tighter connection between lg k, 1/λmax and theory. This might partly reflect the advantage of standardized protocols, avoiding the inter-laboratory variation affecting data assembled from many studies. However, precisely because of the persuasiveness of their elegant study, it seems important to keep up awareness that not everything can be so neatly wrapped up. By the examples in Figs. 5 and 6, I wish to emphasize that there exists real and substantial variation in dark event rates between rod rhodopsins that differ negligibly in λmax. On a general level, this can be seen at a glance in Fig. 4 A from the scatter of k values near 1/λmax ≈ 2 × 106 m–1, i.e., for rods having typical rod λmax values ≈ 500 nm.
Fig. 5.
Differing rates of discrete dark events in rhodopsin rods of a toad and a frog with virtually identical absorption spectra (λmax ≈ 502–503 nm; [6]): (A) from a rod of Bufo marinus [26]; (B) from an A1 rhodopsin rod of Ran-a catesbeiana [57]. All records are displayed on the same time scale, shown below panel (A); current scale bars on the left. Temperature was 22 °C for Bufo and 17°C for Rana. In both (A) and (B), the three top traces show recordings in complete darkness. In Bufo (A), discrete dark events (“bumps”) can be clearly discerned as well as continuous noise. The continuous noise is also of biological origin, as shown by the bottom trace where all biological noise has been eliminated by keeping the rod saturated with strong background light. In Rana (B), only continuous noise but no clear discrete events can be seen during nearly 20 minutes of dark recording. This is not due to poor preparation or recording, as shown by two epochs of light responses (bottom traces) to flashes of nominal light intensity 1.25 R* delivered at 10 s intervals. The SQR amplitude is about 0.4 pA as determined both from the nominal light intensity (0.43 pA) and from the variance-to-mean ratio of the flash responses (0.39 pA). The first flash epoch was recorded before the dark recordings, the second between the second and third dark record.
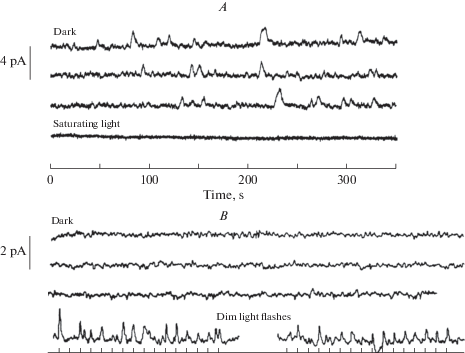
Fig. 6.
Dark event rates in 5 salamander rods as a function of the estimated fraction of A2 pigment in the rods before and after replacement of a significant part of the (native) A2 chromophore by A1, plotted on linear (A) and logarithmic (B) scales. The linear regression of event rate (Y) on A2 content (X) (Y = 0.0009 + 0.318X, straight line) extrapolates (for X = [A2] = 0) to one event per 18 minutes among the ca. 3.8 × 109 A1 rhodopsin molecules of the mean recorded rod volume. The rate per molecule of visual pigment is similar to that in A1 rods of Rana catesbeiana (cf. Fig. 5 B,where there is not a single dark event during nearly 20 minutes of recording). Reproduced from [67].
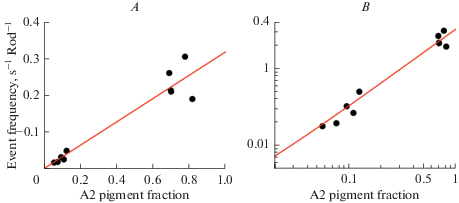
Fig. 5 shows dark current recordings from rods of two anuran species with roughly the same λmax (502–503 nm). Panel A shows the iconic first example of dark rod events published by Baylor et al. (1980) [26]. In the 1050 seconds of recording from a Bufo marinus rod, a fair number of discrete “bumps” (some 20) can be identified by eye. This and similar recordings from 9 cells, analyzed by several different methods in their study, indicated 0.02–0.03 events per rod per second. Panel B shows a recording from an A1 rod of Rana catesbeiana [57]. In ca 20 minutes, not a single discrete bump can be seen (implying a rate of <0.0008 per second), nor did more sophisticated analyses indicate any. Two epochs of responses to light flashes delivering ca 1 photoisomerization on average are shown below the “dark” traces. The first of these was taken before all the dark epochs, the second between the two latter ones, showing that the absence of dark events is not due to SQRs being undetectable in this rod and recording. Such total silence in darkness was observed in 3 other rods out of 5 studied. In one rod a rate of 0.006 per second was determined from the current probability density histogram, and in a sixth rod recorded after the publication of [57], there was one event distinctly identified by eye in a ca. 1000 second epoch (implying 0.001 per second, or 2.5 × 10–13 events per pigment molecule per second, R* s–1). By any statistics, these results are incompatible with those from Bufo marinus, and even in the 2 out of 6 rods where any dark events at all were detected, it is doubtful whether they originate in the A1 pigment. Rana catesbeiana, as opposed to Bufo marinus, uses the inherently less stable A2 chromophore during the larval stage and even in the adult stage in the dorsal retina [65], but minimal amounts in other parts of the retina cannot be excluded. (It should be noted that SQRs produced by A1 and A2 pigments cannot be distinguished [66]). A natural hypothesis would be that the Rana opsin has evolved to limit noise when collaborating with A2, and that this results in exceptional stability of the A1 version of the pigment. Even a minute fraction of A2 remaining may significantly increase the rate of dark events. P. Ala-Laurila et al. [67] tested the relative noise contribution of A1 and A2 pigment systematically in experiments on rods of larval tiger salamander (Ambystoma tigrinum), where the native pigment is almost 100% A2. They measured changes in dark event rates upon replacing most of the A2 by A1, and plotted the rates as function of remaining A2 (Fig. 6). The dark events decreased linearly with decreasing A2 fraction, extrapolating to a rate of 0.0009 events per rod per second (~2.4 × 10–13 R* s–1) for zero A2 (pure A1). This suggests that A1 pigments of Ambystoma tigrinum (λmax = 502 nm) and Rana catesbeiana (λmax = 502 nm) have roughly the same, extremely low thermal activation rates, which are more than one order of magnitude lower than those in Bufo marinus (λmax = 503 nm) ([26]: 1.2 × 10–11 R*s–1; [39]: 3.2 × 10–12 R* s–1) and in Bufo bufo (λmax = 502 nm) ([68]: 5.4 × 10–12 R* s–1; [34]: 8.4 × 10–12 R* s–1). (All values refer to rates temperature-corrected to 21°C.) With respect to the existence of species differences, it may further be noted that Luo et al. [39] found a 16-fold higher temperature-corrected activation rate per molecule of visual pigment in mouse rods compared with Bufo marinus rods. A priori, toad and mouse pigments are expected to be roughly similar, and if anything, a possible difference would be expected to go in the opposite direction (mouse rod λmax = 497 nm [69]).
Thus there can be little doubt that true interspecies variation exists around the average functional relationships of λmax, k and Ea (Fig. 1). However, besides species differences and “acceptable” random differences between results from different laboratories, there are cases where results on the same photoreceptor species differ to a degree for which there is no clear explanation. It is tempting to think that there could be polymorphisms between populations/strains of the same species used in different laboratories. These amphibians, unlike laboratory rodents, have not been bred for global standardization of strains. For example, significant spectral polymorphism (not due to varying A2 admixture) has been found between individuals of the common frog Rana temporaria, with rod λmax varying by 8 nm, far more than normal experimental variation [70]. Above, I have also referred to the significant differences in Ea and k values of Bufo marinus reported in [39] compared with those in [45] (Ea) and [26] (k).
A major unresolved conflict of this kind concerns the thermal stability of the blue-sensitive pigment of Bufo “green” rods (with λmax ≈ 432–433 nm, [6]). Whereas G. Matthews [54] originally reported an unexpectedly high dark event rate, 4 times higher than in the “red” rods (see above), D.G. Luo et al. [39] found an event rate 100 times lower than in the “red” rods. The high value seemed to make sense when it was shown that the anuran green-rod pigment is an SWS2 cone pigment [55]. Cone pigments are generically less stable by 2–3 orders of magnitude [71–73, 39, 42 ], but the most short-wavelength-sensitive ones may approach the stability of rod pigments [74, 75]. The recent discovery of a stabilizing mutation in the anuran green-rod SWS2 pigment [56] might give reason to expect it to be particularly silent even compared with rod pigments, but really there is no easy explanation for the large discrepancy of the estimates in [54] and [39]. This question certainly merits further investigation.
TUNING OF SPECTRAL ABSORBANCE AND THERMAL STABILITY BY THE OPSIN
Over 30 years, a huge literature has accumulated on how amino acid residues in the opsin tune the chromophore for diffent spectral absorbances in single species and across species (e.g. [76–80]). On a general level, the results exemplify the “multiple realizability” of function in complex biological systems, meaning in this case that (for practical purposes) identical spectral absorbance can be achieved by different combinations of amino acids in critical positions. This also makes it seem likely that similar spectra can be associated with different thermal properties, and that mutations affecting spectral and thermal properties may be independent targets of natural selection. Recently there has been increasing interest specifically in the tuning of thermal stability [56, 81]. Earlier, N. Fyhrquist et al. [82] sequenced the rod opsins of the anuran species presented above as examples where the same λmax is associated with different dark event rates: Rana catesbeiana (plus two other Rana species, as well as Xenopus laevis) vs. the two Bufo species. Although it was not possible to identify unique residues for stability, among sixteen non-conserved substitutions and six involving gain/loss of hydroxyl groups, a few clear contrasts between Bufo and Rana were found. Some of these were shared by the Rana opsins and the Xenopus laevis opsin (which also has to collaborate with the A2 chromophore). The resolution of such studies have improved since then. In [81], two residues were identified that explain at least some of the generically increased stability of rod compared with cone pigments, and in [56] a single threonine at position 47 was identified as responsible for the rod-like stability of the anuran (in contrast to the urodelan) blue-cone pigment (SWS2). The green rods equipped with this stabilized pigment allow frogs to make blue/green wavelength discriminations at the absolute threshold of vision [83].
These developments enable us to address new evolutionary questions. Does the rarity of strongly stabilized rod pigments like those of Rana catesbeiana and Ambystoma tigrinum (Figs. 5 and 6) indicate that pigment noise in e.g. Bufo and other typical vertebrate rods is already driven to an acceptably low level, given the presence of other noise sources? In other words, is the extreme silence of Rana and Ambystoma A1 pigments mainly a side effect of having to limit the noisiness of the A2 pigment? Could there be mutations making pigments with λmax beyond the long-wavelength limit of ca. 630 nm found in nature stable enough to be useful? On a different track, it is interesting to follow the engineering of microbial (“type 1”) rhodopsins with the purpose of developing improved tools for optogenetics, for example, by moving their spectral absorbance further into the infrared [84–87].
Список литературы
Hargrave P.A. Rhodopsin chemistry, structure and topography. Progress in Retinal Res. 1: 1–51. 1982.
Wald G. Carotenoids and the visual cycle. J. Gen. Physiol. 19: 351–371. 1935.
Bridges C.D.B. The rhodopsin-porphyropsin visual system. In: Handbook of Sensory Physiology VII/1: Photochemistry of Vision (ed. H.J.A. Dartnall). 417–480. Berlin. Springer-Verlag. 1972.
Provencio I., Loew E.R., Foster R.G. Vitamin A2-based visual pigments in fully terrestrial vertebrates. Vision Res. 32: 2201–2208. 1992.
Enright J.M., Toomey M.B., Sato S.-Y., Temple S.E., Allen J.R., Fujiwara R., Kramlinger V.M., Nag L.D., Johnson K.M., Xiao Y., How M.J., Johnson S.L., Robert, N.W., Kefalov V.J., Guengerich F.P., Corbo J.C. Cyp27c1 red-shifts the spectral sensitivity of photoreceptors by converting vitamin A1 into A2. Current Biol. 25: 3048–3057. 2015.
Govardovskii V.I., Fyhrquist N., Reuter T., Kuzmin D.G., Donner K. In search of the visual pigment template. Visual Neurosci. 17: 509–528. 2000.
Dartnall H.J.A., Lythgoe J.N. The spectral clustering of visual pigments. Vision Res. 5: 81–100. 1965.
Hárosi F.I. An analysis of two spectral properties of vertebrate visual pigments. Vision Res. 34: 1359–1367. 1994.
Autrum H. Über kleinste Reize bei Sinnesorganen. Biol. Zentralblatt. 66: 209–236. 1943.
Barlow H.B. Retinal noise and absolute threshold. J. Opt. Society of America. 46: 634–639. 1956.
Denton E.J., Pirenne M.H. The absolute sensitivity and funcional stability of the human eye. J. Physiol. 123: 417–442. 1954.
Yau K.W., Lamb T.D., Baylor D.A. Light-induced fluctuations in membrane current of single toad rod outer segments. Nature. 269: 78–80. 1977.
Neher E., Sakmann B. Single-channel currents recorded from membranes of denervated frog muscle fibres. Nature. 260: 799–802. 1976.
Baylor D.A., Lamb T.D., Yau K.-W. Responses of retinal rods to single photons. J. Physiol. 288: 613–634. 1979.
Pugh E.N., Lamb T.D. Amplification and kinetics of the activation steps in phototransduction. Biochim. Biophys. Acta. 1141: 111–149. 1993.
Leskov I.B., Klenchin V.A., Handy J.W., Whitloc G.G., Govardovskii V.I., Bownds M.D., Lamb T.D., Pugh E.N., Jr., Arshavsky V.Y. The gain of rod phototransduction: reconciliation of biochemical and electrophysiological measurements. Neuron. 27: 525–537. 2000.
Arshavsky V.Y., Burns M.E. Current understanding of signal amplification in phototransduction. Cellular Logistics. 4: e29390 DOI:. 2014.https://doi.org/10.4161/cl.29390
Yue W.W.S., Silverman D., Ren X., Frederiksen R., Sakai K., Yamashita T., Shichida Y., Cornwall M.C., Chen J., Yau K.-W. Elementary response triggered by transducin in retinal rods. PNAS. 116: 5144–5153. 2019.
Rieke F., Baylor D.A. Origin of reproducibility in the responses of retinal rods to single photons. Biophysi. J. 75: 1836–1857. 1998.
Whitlock G.G., Lamb T.D. Variability in the time course of single photon responses from toad rods: termination of rhodopsin’s activity. Neuron. 23: 337–351. 1999.
Field G.D., Rieke, F. Mechanisms regulating variability of the single photon responses of mammalian rod photoreceptors. Neuron. 35: 733–47. 2002.
Hamer R.D., Nicholas S.C., Tranchina D., Liebman P.A., Lamb T.D. Multiple steps of phosphorylation of activated rhodopsin can account for the reproducibility of vertebrate rod single-photon responses. J. Gen. Physiol. 122: 419–444. 2003.
Doan T., Mende A., Detwiler P.B., Chen J., Rieke F. Multiple phosphorylation sites confer reproducibility of the rod’s single-photon responses. Science. 313: 530–533. 2006.
Krispel C., Chen D., Melling N., Chen Y.-J., Martemyanov K.A., Quillinan N., Arshavsky V.Y., Wensel T.G., Chen C.-K., Burns M.E. RGS expression rate-limits recovery of rod photoresponses. Neuron. 51: 409–416. 2006.
Lamb T.D., Kraft T.W. Quantitative modeling of the molecular steps underlying shut-off of rhodopsin activity in rod phototransduction. Mol. Vision. 22: 674–696. 2016.
Baylor D.A., Matthews G., Yau K.W. Two components of electrical dark noise in toad retinal rod outer segments. J. Physiol. 309: 591–621. 1980.
Hubbard R. The stereoisomerization of 11-cis retinal. J. Biol. Chem. 241: 1814–1818. 1966.
Cooper A. Energy uptake in the first step of visual excitation. Nature. 282: 531–533. 1979.
Lythgoe R.J., Quilliam J.P. The thermal decomposition of visual purple. J. Physiol. 93: 24–38. 1938.
St. George R.C.C. The interplay of light and heat in bleaching rhodopsin. J. Gen. Physiol. 35: 495–517. 1952.
Barlow R.B.Jr., Birge R.R., Kaplan E., Tallent J.R. On the molecular origin of photoreceptor noise. Nature. 366: 64–66. 1993.
Yeandle S. Evidence of quantized slow potentials in the eye of Limulus. Am. J. Ophthalmol. 46: 82–87. 1958.
Yeandle S., Spiegler J.B. Light-evoked and spontaneous discrete waves in the ventral nerve photoreceptor of Limulus. J. Gen. Physiol. 61: 552–571. 1973.
Firsov M.L., Donner K., Govardovskii V.I. pH and rate of ‘‘dark’’ events in toad retinal rods: test of a hypothesis on the molecular origin of photoreceptor noise. J. Physiol. 539: 3–46. 2002.
Sampath A.P., Baylor D.A. Molecular mechanism of spontaneous pigment activation in retinal cones. Biophysic. J. 83: 184–193. 2002.
Bókkon I., Vimal R.L.P. Retinal phosphenes and discrete dark noises in rods: a new biophysical framework. J. Photochem. Photobiol. B 96: 255–259. 2009.
Govardovskii V.I., Astakhova L.A., Rotov A.Yu., Firsov M.L. Rejection of the biophoton hypothesis on the origin of photoreceptor dark noise. J. Gen. Physiol. 151: 887–897. 2019.
Field G.D., Rieke F. Nonlinear signal transfer from mouse rods to bipolar cells and implications for visual sensitivity. Neuron. 34: 773–785. 2002.
Luo D.G., Yue W.W., Ala-Laurila P.,Yau K.W. Activation of visual pigments by light and heat. Science. 332: 1307–12. 2011.
Schick G.A., Cooper T.M., Holloway R.A., Murray L.P., Birge R.R. Energy storage in the primary photochemical events of rhodopsin and isorhodopsin. Biochemistry. 26: 2556–2562. 1987.
Tallent J.R., Hyde E.W., Findsen L.A., Fox G.C., Birge R.R. Molecular dynamics of the primary photochemical event in rhodopsin. J. Am. Chem. Society. 114: 1581–1592. 1992.
Ala-Laurila P., Donner K., Koskelainen A. Thermal activation and photoactivation of visual pigments. Biophys. J. 86: 3653–3662. 2004.
Hinshelwood C.N. The kinetics of chemical change in gaseous systems. Clarendon Press. Oxford. UK. 1933.
Lewis P.R. A theoretical interpretation of spectral sensitivity curves at long wavelengths. J. Physiol. 130: 45–52. 1955.
Ala-Laurila P., Saarinen P., Albert R., Koskelainen A., Donner K. Temperature effects on spectral properties of red and green rods in toad retina. Visual Neurosci. 19: 781–792. 2002.
Okada T., Ernst O.P., Palczewski K., Hofmann K.P. Activation of rhodopsin: new insights from structural and biochemical studies. Trends Biochem. Sci. 26: 318–324. 2001.
Mathies R.A. Photons, femtoseconds and dipolar interactions: a molecular picture of the primary events in vision. Novartis Found. Symp. 224: 70–84. 1999.
Schoenlein R.W., Peteanu L.A., Mathies R.A., Shank C.V. The first step in vision: femtosecond isomerization of rhodopsin. Science. 254: 412–415. 1991.
Gozem S., Schapiro I., Ferré N., Olivucci M. On the molecular mechanism of thermal noise in rod photoreceptors. Science. 337: 1225–1228. 2012.
Polli D., Altoè P., Weingart O., Spillane K., Manzoni C., Brida D., Tomasello G., Orlandi G., Kukura P., Mathies R.A., Garavelli M., Cerullo G. Conical intersection dynamics of the primary photoisomerization event in vision. Nature. 467: 440–443. 2010.
Luk H.L., Bhattacharyya N., Montisci F., Morrow J.M., Melaccio F., Wada A., Sheves M., Fanelli F., Chang B.S.W., Olivucci M. Modulation of thermal noise and spectral sensitivity in Lake Baikal cottoid fish rhodopsins. Scient. Rep. 6:38425. 2016. https://doi.org/10.1038/srep38425
de Vries H. Comment to Dr. Wald’s lecture. Document. Ophthalmol. 3: 137. 1949.
Barlow H.B. Purkinje shift and retinal noise. Nature. 179: 255–256. 1957.
Matthews G. Dark noise in the outer segment membrane current of green rod photoreceptors from toad retina. J. Physiol. 349: 607–618. 1984.
Hisatomi O., Takahashi Y., Taniguchi Y., Tsukahara Y., Tokunaga F. Primary structure of a visual pigment in bullfrog green rods. FEBS Letters. 447: 44–48. 1999.
Kojima K., Matsutania Y., Yamashita T., Yanagawa M., Imamoto Y., Yamano Y., Wada A., Hisatomi O., Nishikaw, K., Sakurai K., Shichida Y. Adaptation of cone pigments found in green rods for scotopic vision through a single amino acid mutation. PNAS. 114: 5437–5442. 2017.
Donner K., Firsov M.L., Govardovskii V.I. The frequency of isomerization-like"dark" events in rhodopsin and porphyropsin rods of the bull-frog retina. J. Physiol. 428: 673–692. 1990.
Firsov M.L., Govardovskii V.I. Dark noise of visual pigments with different absorption maxima. Sensornye Sistemy. 4: 25–34. 1990. (In Russ).
Stiles W.S. The physical interpretation of the spectral sensitivity curve of the eye. In: Transactions of the Optical Convention of the Worshipful Company of Spectacle Makers. Spectacle Makers’ Co. London. UK. 97–107. 1948.
Srebro R. A thermal component of excitation in the lateral eye of Limulus. J. Physiol. 187. 417–425. 1966.
Koskelainen A., Ala-Laurila, P., Fyhrquist N., Donner K. Measurement of thermal contribution to photoreceptor sensitivity. Nature. 403: 220–223. 2000.
Ala-Laurila P., Albert R-J., Saarinen P., Koskelainen A., Donner K. The thermal contribution to photoactivation in A2 visual pigments studied by temperature effects on spectral properties. Visual Neurosci. 20: 411–419. 2003.
Pahlberg J., Lindström M., Ala-Laurila P., Fyhrquist-Vanni N., Koskelainen A., Donner K. The photoactivation energy of the visual pigment in two spectrally different populations of Mysis relicta (Crustacea, Mysida). J. Compar. Physiol. A 191: 837–844. 2005.
Ala-Laurila P., Pahlberg J., Koskelainen A., Donner K. On the relation between the photoactivation energy and the absorbance spectrum of visual pigments. Vision Res. 44: 2153–2158. 2004.
Reuter T.E., White R.H., Wald G. Rhodopsin and porphyropsin fields in the adult bullfrog retina. J. Gen. Physiol. 58: 351–371. 1971.
Firsov M.L., Govardovskii V.I., Donner K. Response univariance in bull-frog rods with two visual pigments. Vision Res. 34: 839–847. 1994.
Ala-Laurila P., Donner K., Crouch R.K., Cornwall M.C. Chromophore switch from 11-cis dehydroretinal (A2) to 11-cis-retinal (A1) decreases dark noise in salamander red rods. J. Physiol. 585: 57–74. 2007.
Fyhrquist N., Govardovskii V.I., Leibrock C., Reuter T. Rod pigment and rod noise in the European toad Bufo bufo. Vision Res. 38: 483–486. 1998.
Smeds L., Takeshita D., Turunen T., Tiihonen J., Westö J., Martyniuk N., Seppänen A., Ala-Laurila P. Paradoxical rules of spike train decoding revealed at the sensitivity limit of vision. Neuron. 104: 576–587. 2019.
Bowmaker J.K., Loew E.R., Liebman P.A. Variation in the λmax of rhodopsin from individual frogs. Vision Res. 15: 997–1003. 1975.
Kefalov V., Fu Y., Marsh-Armstrong N., Yau K.-W. Role of visual pigment properties in rod and cone phototransduction. Nature. 425: 526–531. 2003.
Sakura K., Onishi A., Imai H., Chisaka O., Ueda Y., Usukura J., Nakatani K., Shichida Y. Physiological Properties of Rod Photoreceptor Cells in Green-sensitive Cone Pigment Knock-in Mice. J. Gen. Physiol. 130: 21–40. 2007.
Fu Y., Kefalov V., Luo D.G., Xue T., Yau K.W. Quantal noise from human red cone pigment. Nature Neurosci. 11: 565–71. 2008.
Rieke F., Baylor D.A. Origin and functional impact of dark noise in retinal cones. Neuron. 26: 181–186. 2000.
Shi G., Yau K.-W., Chen J., Kefalov V.J. Signaling properties of a short-wave cone visual pigment and its role in phototransduction. J. Neurosci. 27: 10084–10093. 2007.
Nathans J. Determinants of visual pigment absorbance: role of charged amino acids in the putative transmembrane segments. Biochemistry. 29: 937–942. 1990.
Yokoyama S. Amino acid replacements and wavelength absorption of visual pigments in vertebrates. Mol. Biol. Evol. 12: 53–61. 1995.
Yokoyama S., Yang H., Starmer W.T. Molecular basis of spectral tuning in the red- and green-sensitive (M/LWS) pigments in vertebrates. Genetics. 179: 2037–2043. 2008.
Hunt D.M., Dulai K.S., Partridge J.C., Cottrill P., Bowmaker J.K. The molecular basis of a spectral tuning of rod visual pigments in deep-sea fish. J. Exper. Biol. 204: 3333–3344. 2001.
Hunt D.M., Carvalho L.S., Cowing J.A., Davies W.L. Evolution and spectral tuning of visual pigments in birds and mammals. Philosoph. Transactions of the Royal Soc. B 364: 2941–2955. 2009.
Yanagawa M., Kojima K., Yamashita T., Imamoto Y., Matsuyama T., Nakanishi T., Yamano Y., Wada A., Sako Y., Shichida Y. Origin of the low thermal isomerization rate of rhodopsin chromophore. Scient. Rep. 5: 11081. DOI: . 2015https://doi.org/10.1038/srep11081
Fyhrquist N., Donner K., Hargrave P.A., McDowell J.H., Popp M.P., Smith W.C. Rhodopsins from three frog and toad species: Sequences and functional comparisons. Exp. Eye Res. 66: 295–305. 1998.
Yovanovich C.A.M., Koskela S.M., Nevala N., Kondrashev S.L., Kelber A., Donner K. The dual rod system of amphibians supports colour discrimination at the absolute visual threshold. Philos. Transactions of the Royal Society. Biol. Sci. 372: 1717–1726. 2017.
McIsaac R.S., Engqvist M.K., Wannier T., Rosenthal A.Z., Herwig L., Flytzani N.C., Imasheva E.S., Lanyi J.K., Balashov S.P., Gradinaru V., Arnold F.H. Directed evolution of a far-red fluorescent rhodopsin. PNAS. 111: 13034–13039. 2014.
McIsaac R.S., Bedbrook C.N., Arnold F.H. Recent advances in engineering microbial rhodopsins for optogenetics. Current Opinion in Struct. Biol. 33: 8–15. 2015.
Ganapath S., Venselaar H., Chen Q., de Groot H.J.M., Hellingwerf K.J., de Grip W.J. Retinal-based proton pumping in the near infrared. J. Am. Chem. Society. 139: 2338–2344. 2017.
Ganapathy S., Kratz S., Chen Q., Hellingwerf K.J., de Groot H.J.M., Rothschild K.J., de Grip W.J. Red-shifted and near-infrared active analog pigments based upon archaerhodopsin-3. Photochem. and Photobiol. 95: 959–968. 2019.
Дополнительные материалы отсутствуют.
Инструменты
Российский физиологический журнал им. И.М. Сеченова