Ботанический журнал, 2019, T. 104, № 10, стр. 1598-1609
LABILE NITROGEN AVAILABILITY IN SOIL INFLUENCES THE EXPRESSION OF WOOD PATTERN IN KARELIAN BIRCH
N.A. Galibina 1, *, L.L. Novitskaya 1, K.M. Nikerova 1, **, E.V. Moshkina 1, Yu.L. Moshchenskaya 1, M.N. Borodina 1, I.N. Sofronova 1, N.N. Nikolaeva 1
1 Forest Research Institute, Karelian Research Centre, Russian Academy of Sciences
185910 Petrozavodsk, Pushkinskaya Str., 11, Karelia, Russia
* E-mail: galibina@krc.karelia.ru
** E-mail: knikerova@yandex.ru
Поступила в редакцию 8.04.2019
После доработки 2.10.2019
Принята к публикации 8.10.2019
Аннотация
We investigated the effect of soil fertility (especially nitrogen level) on the expression of patterned wood in Karelian birch (Betula pendula var. carelica). We carried out soil analysis in the middle taiga subzone of Karelia in the Kondopoga, Pryazha and Medvezhiegorsk Districts, where Karelian birch trees with varying wood figure intensities were growing. The cambial zone of the sample trees was studied for the activity of nitrate reductase (NR) – a key enzyme for the assimilation of nitrates, and for sucrose synthase (SUS) and apoplastic invertase (ApInv) activities. It was found that the formation of patterned wood is promoted by a reduction in SUS activity (decrease in the amount of xylem vessels and fibrous elements) and an increase in the activity of ApInv (increase in the amount of parenchyma) in the area of xylem growth and development. Normal level of nitrogen availability (~80–100 kg/ha) provides for normal plant growth, and high SUS and ApInv activities in the xylem, and ApInv activity in the phloem. Some reduction in soil fertility (nitrogen within ~70 kg/ha) leads to a decrease in SUS activity together with a rise in ApInv activity and an increase in the amount of dark-colored inclusions in the wood. Simultaneous reduction of the nitrogen level and increase of the phosphorus content causes an increased assimilation of nitrates in trunk tissues and elevated NR. This ratio of nitrogen and phosphorus results in an escalation of competition for photoassimilates between the process of patterned wood formation (sucrose metabolized via the apoplastic pathway) and plant growth (sucrose utilized in the xylem for cell wall formation). This study has shown that the availability of nitrogen and its ratio to the phosphorus level in the soil influence the expression of figure in the wood in Karelian birch, as indicated by variations in the activity of enzymes. An increased supply of nitrate nitrogen to a plant entails a rise in NR activity, which negatively affects the formation of patterned wood.
Abnormal patterned wood of Karelian birch (Betula pendula Roth var. carelica (Mercl.) Hämet-Ahti) is in great demand in markets around the world. The pattern is generated by the deposition of dark-colored parenchymatous inclusions of various shapes (Fig. 1) (Novitskaya, 1998). The differentiating cambial derivatives retain the protoplast and are transformed into storage parenchyma cells (Novitskaya, 1998, 2008; Novitskaya, Kushnir, 2006). Cambial cells are in donor-acceptor relationship with foliage because cambium is a heterotrophic tissue whose activity depends on the influx of carbohydrate photoassimilates (sugars) from leaves. Sucrose is transported from leaves to trunk tissues, and this is the main transport form of sugars in birch (Novitskaya et al., 2015). Sucrose is metabolically inert and is utilized in sink tissues only after being metabolized by invertase (ApInv) and sucrose synthase (SUS) (Sturm, Tang, 1999; Koch, Zeng, 2002, etc.).
Fig. 1.
External appearance (a–d), debarked trunk surface (e–h), and transverse sections (i–l) of figured Karelian birch plants from plot 1 (a, e, i), plot 2 (b, f, j), plot 3 (c, g, k), and plot 4 (d, h, l). Numbers in parentheses for each plot are the reserves of major nutrients (N, P, K) in the upper 25-cm soil layer, kg/ha. Numbers above the photographs of sawn sections represent the percent area of parenchyma inclusions inside a 5 cm2 rectangle in the periphery of the section. Bar – 1 cm.
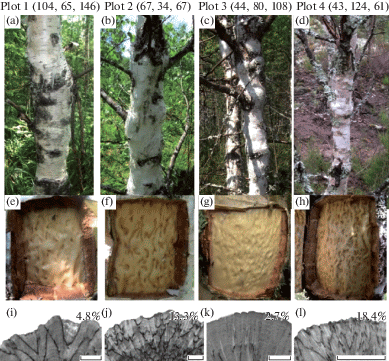
Our previous studies (Galibina et al., 2015a, b) showed that Karelian birch with abnormal patterned wood differs from silver birch (B. pendula var. pendula) with normal straight-grained wood in the pathways of sucrose metabolism in trunk tissues during period of cambial activity. In silver birch, sucrose is actively utilized in the cambial zone and differentiating xylem by SUS, the enzyme that cleaves sucrose into UDP-glucose and fructose.
In contrast, Karelian birch features a suppression of SUS activity in the zone of xylem formation (Galibina et al., 2015a; Moshchenskaya et al., 2017), resulting in excessive sucrose accumulation in parenchyma cells and its transfer to the apoplast, where sucrose is cleaved by apoplastic invertase to glucose and fructose (Galibina et al., 2015b). When these hexoses are returned to parenchyma cells, they promote the synthesis of reserve metabolites, enhancing the transformation of cambial derivatives to storage parenchyma cells, which form a structural background for the patterned wood (Novitskaya et al., 2016). A variation in the ratio of SUS to ApInv activities underlies the great variation in the degree of wood patterning observed in Karelian birch trees. In particular, the SUS form associated with plasmalemma is a component of the cellulose synthase complex and supplies UDP-glucose for cellulose synthesis (Amor et al., 1992; Ruan et al., 2003; Fujii et al., 2010; Song et al., 2010, etc.).
Although the ability to form patterned wood is inherited (Novitskaya, 2008), it does not appear in all Karelian birch trees. The plants with abnormal wood structure commonly constitute 50–70% of the total progeny, and they vary widely in the expression of wood figure (Lyubavskaya, 1978; Evdokimov, 1989). This fact influences the commercial value of the timber.
There are many environmental factors that can affect wood quality. We hypothesized that changes in nitrogen metabolism parameters can affect wood quality by influencing carbohydrate metabolism. There is significant evidence of the effects of nitrogen nutrition on carbohydrate metabolism in plants (Gordon et al., 1999, 2002; Chikov et al., 2003, 2004; Batasheva, 2006; Bruskova et al., 2009; Nikitin et al., 2010; Nikitin, Izmailov, 2016, etc.). In general, plants absorb nitrogen from the soil mainly as ${\text{NH}}_{4}^{ + }$ and ${\text{NO}}_{3}^{ + }$. Of these two ions, the latter one is involved in the regulation of plant metabolism and development. The nitrate ion can induce both the NO3- assimilatory pathway and the reprogramming of carbon metabolism to provide reductants and C skeletons for this pathway (Forde, 2002).
We investigated the effect of exogenous nitrate on SUS and ApInv activities in the trunks of silver birch and Karelian birch during the period of cambial activity (Galibina et al., 2016). In silver birch, the application of nitrate promoted sucrose utilization by SUS, which, as has been observed previously, correlates with the growth of normal wood and increase in the xylem cellulose content. In Karelian birch xylem, nitrates induced a decline in the activity of both enzymes resulting in decrease in wood growth due to SUS decline, and reduction of parenchyma amount, i.e. normalization of wood structure due to ApInv decline.
The aim of this study is to clarify the effect of soil conditions (especially soil nitrogen levels) on the activity of nitrate reductase (NR, EC 1.7.1.1, a key enzyme in nitrate assimilation), SUS and ApInv in the secondary xylem and secondary phloem of Karelian birch, and on the expression of abnormal patterning in its wood. To this end, we analyzed soils from areas where Karelian birch trees with varying degrees of wood figure were growing.
MATERIAL AND METHODS
Description of sites
We studied Karelian birch plants growing in the middle taiga subzone (62°16′ N and 33°59′ E) in the Kondopoga, Pryazha and Medvezhiegorsk Districts of Karelia, northwestern Russia. These districts belong to the Baltic physiographic domain of the boreal zone. The area is situated in the southeastern fringes of the Baltic (Fennoscandian) Crystalline Shield. The plants were sampled from artificial plantations established in former clearcuts by re-planting saplings grown from selected seeds. The sample plots differ in the plant cover and soil properties. Soils of the study area were identified according to the International classification system of the World Reference Base (IUSS Working Group WRB, 2015).
The first plot (1) was a 2.6 ha area in the Kondopoga District. The terrain is flat, with normal moisture conditions. The soil is skeletal Entic Podzol. Understory vegetation consists of Vaccinium myrtillus, Geranium sylvaticum, Trientalis europaea, Poaceae spp., Solidago virgaurea, Convallaria majalis, Polypodiophyta, Anthriscus sylvestris. Grasses (Poaceae) dominate in the herbaceous layer. Tree age at the time of sampling was 23 years (Fig. 1a). The trees were robust.
The second plot (2) was a 2 ha area in the Medvezhiegorsk District. The soil is Gleyic Podzol. The plot is located in a low-lying part of the plantation with abundant moisture. Understory vegetation consists of Polytrichum commune, Vaccinium vitis-idaea (rare), Epilobium angustifolium. The herbaceous layer was dominated by Vaccinium vitis-idaea. Tree age at the time of sampling was 25 years (Fig. 1b). The trees were robust.
The third plot (3) is a 0.8 ha area in the Pryazha District. The soil is Entic Podzol. The plot is located in a low-lying part of the plantation. The site has a complex hilly topography with abundant leaf litter. Understory vegetation consists of Calluna vulgaris, Vaccinium vitis-idaea, Pleurozium schreberi, Cladonia, and is dominated by Vaccinium vitis-idaea and Pleurozium schreberi. The age of the trees was 23 years (Fig. 1c). The trees were lower and thinner than could be expected at this age.
The fourth plot (4) is a 1.2 ha area in the Pryazha District. The soil is Entic Podzol. The plot is located on the top of a hill. The organogenic horizon is mainly represented by previous year’s litter. Understory vegetation consists of Calluna vulgaris, Vaccinium vitis-idaea, mosses (Pleurozium, Polytrichum) and lichens (Cladonia), and is dominated by Calluna vulgaris and Pleurozium schreberi. The age of the trees was 23 years. The plants were in suppressed state, 20% of the trees were weakened (Fig. 1d).
Plant material and sampling
We studied high-stemmed Karelian birch plants with patterned wood. All the plants were raised from seeds obtained by controlled pollination of Karelian birch plus trees, which had a pronounced wood figure. We selected 5 trees in each plot.
The plants were grouped by the extent of patterned wood (Fig. 1e–h) using a technique proposed earlier (Galibina et al., 2016). The expression of wood figure was estimated from trunk cross-sections. One tree from each group of trees was cut down after doing trunk cross-sections. The cross-sections were made by sawing the trunks directly above the ‘window’ (debarked portion of the trunk) in which the number of depressions (pits) on wood surface was counted to estimate degree of wood patterning. The sawn sections were polished and photographed. A rectangular area 2×3 cm, with the longer side oriented along growth rings, was chosen on each cross-section closer to the latest annual rings near the debarked portion for doing biochemical tests. To estimate the density of the figured pattern in the wood, the percentage of dark parenchymatous inclusions in these rectangular areas was measured using the Morphology 5 software (Videotest, Russia) (Fig. 1i–l). According to these measurements, there were several groups. Each group was scored from 1 to 3. The first group (scored 1) included patterned plants from plot 3 (Fig. 1g, k), the second one (scored 2) – patterned plants from plot 1 (Fig. 1e, i), and the third group (scored 3) – patterned plants from plot 2 (Fig. 1f, j) and plot 4 (Fig. 1h, l).
The cambium of silver birch in Karelia is active from the second decade of June to the first decade of August (Novitskaya et al., 2008). The samples were collected on 01–03.07.2016.
‘Windows’ of 60 cm2 were excised on birch trunks, and bark was separated from wood to sample xylem and phloem tissues. The xylem tissues comprising xylem mother cells and outer layers of the current-year xylem growth were scraped off the wood surface. From the inner surface of the bark we excised phloem tissues comprising the cambial zone, the conducting phloem, and the innermost layers of non-conducting phloem. The samples of trunk tissues were examined under a light microscope. We froze all plant material in liquid nitrogen immediately and kept it in a low temperature freezer at –70°C.
Soil analyses
Samples were collected from soil genetic horizons and analyzed following the techniques commonly used in soil science. Total nitrogen and carbon were determined using ultimate CHNS analyzer (PerkinElmer’s 2400 Series II CHNS/O, USA). The analysis of labile phosphorus was made with the ammonium-molybdate method using spectrophotometer (OKB Spektr SF-2000, Russia). Labile potassium was analyzed in flame atomic spectrophotometer (Shimadzu AA 7000, Japan). Reserves of nitrogen, phosphorus and potassium were calculated for the 0–25 cm soil layer (including the organic horizon with litter) because that is where the main forms of the nutrients available to plants are accumulated. Nutrient reserves determined for individual soils were recalculated for the areas occupied by these soils in the landscape (Bakhmet, 2012).
Biochemical tests
Plant tissues were ground in liquid nitrogen to a uniform mass and homogenized at 4°C in the buffer containing 50 mM HEPES (p 7.5), 1 mM EDTA, 1 mM EGTA, 3 mM DTT, 5 mM MgCl2 and 0.5 mM PMSF. After 20-min extraction, the homogenate was centrifuged at 10 000 g for 20 min (MPW-351R, Poland). The sediment was washed in the buffer thrice. The pooled supernatant and sediment were dialyzed at 4°C for 18–20 h against tenfold diluted homogenization buffer. Free hexoses were undetectable in the enzyme preparations obtained after dialysis. ApInv associated with the cell wall was determined in the sediment, whereas SUS and NR in the supernatant. The incubation medium for NR activity determination contained 50 mM phosphate buffer (pH 7.6), 10 mM KNO3, 0.7 mM NAD(P)H, 0.5 mM EDTA, 0.625 mM cysteine, 25 µM Na2MoO4 (Frieman et al., 1992). The nitrite amount formed during the incubation was determined spectrophotometrically (OKB Spektr SF-2000, Russia) with the Griess reagent. NR activity was determined after incubation at 30°C for 10 min and expressed in nmol of nitrite formed per 1 mg protein (nmol/mg protein). Proteins in the extracts were quantified by the method of Bradford.
The incubation medium for SUS activity determination contained 73 mM Tris-HCl (pH 7.5), 2.5 mM UDP-glucose, 20 mM fructose, 5 mM MgCl2, 3 mM DTT. The SUS activity directed to sucrose synthesis was determined after incubation at 30°C for 30 min. The sucrose amount formed during the incubation was determined spectrophotometrically by ROE method. The SUS activity was expressed in μmol of formed sucrose per 1 mg protein (μmol/mg protein) (Moshchenskaya et al., 2017).
The incubation medium for determining ApInv contained 100 mM acetate buffer (pH 4.7) and 25 mM sucrose. The glucose amount formed during the incubation was determined by the glucose oxidation technique (Glukoza-Agat kit, Russia). The ApInv activity was determined after incubation at 30°C for 30 min and expressed in μmol of degraded sucrose per 1 g fresh weight (μmol/g FW) (Galibina et al., 2015b).
Statistical processing
Statistical processing was carried out in Microsoft Excel and StatGraphics for Windows. The data are shown as arithmetic means from a biological replication of 5 trees in each group in triple analytical replications. The confidence intervals for all values were estimated according to Student’s t-distribution, р < 0.05.
RESULTS
Soil conditions and nutrient requirements of birch
The plots differ in the natural fertility of soil, as evidenced by different reserves of major nutrients in the root layer (Table 1).
Table 1.
Reserves of major nutrients in the upper 25-cm soil layer Таблица 1. Запасы основных питательных веществ в верхнем 25-сантиметровом слое почвы
Plot | N, kg/ha | P, kg/ha | K, kg/ha |
---|---|---|---|
plot 1 | 104 | 65 | 146 |
plot 2 | 67 | 34 | 67 |
plot 3 | 44 | 80 | 108 |
plot 4 | 43 | 124 | 61 |
These data suggest that plot 1 had normal reserves of essential nutrients in labile form required for birch trees; plot 2 was characterized by a reduced nitrogen availability and reduced mineralization under wetter conditions, whereas plot 3 and plot 4 were deficient in nitrogen while phosphorus level was elevated (especially in plot 4).
Morphological and anatomical characteristics of plants
In plot 1 and plot 2, tree trunks were 1.4-fold greater in diameter than those in plot 3 and plot 4 (р = 0.001) (Fig. 1 a–d, 2b). The plants in plot 1 were taller than plants in plot 2 (р = 0.003) (Fig. 2a).
Fig. 2.
Morphological characteristics of plants growing in plots with different soil fertility levels. Bars indicate standard errors.
h – tree height, m (a), d – trunk diameter at 1.3 m above the ground, cm (b).
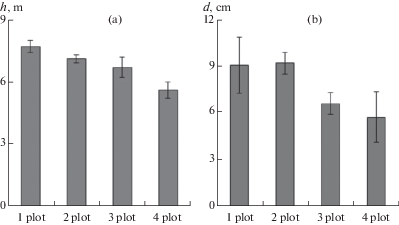
The plants in plot 2 and plot 4 had the higher percentage of dark parenchymatous inclusions in the wood (13.3% and 18.4%, respectively) and, hence, a more expressed figure than the plants in plot 1 and plot 3 (Fig. 1 e–l). The plants in plot 3 had the least intense wood figure (Fig. 1g, k). The differences were statistically significant (p < 0.05).
Thus, only Karelian birch plants in plot 2 had age-appropriate diameter and height and formed the wood with a higher density of the figured pattern.
Nitrate reductase activity
The trees growing in plot 1 had the highest NR activity in the xylem (81 nmol/mg protein), and a lower NR activity in the phloem (23 nmol/mg protein). The same level of NR activity in the phloem coupled with low (9 nmol/mg protein) NR activity in the xylem was found in the trees from the site with lower nitrogen availability (plot 2). NR activity in the phloem of the plants growing under nitrogen deficit (plots 3 & 4) was 3 and 2 times higher, respectively, than in the plants from plot 1. NR activity in their xylem (55 & 60 nmol/mg protein) was lower than that of the plants from plot 1 (Fig. 3).
Fig. 3.
The activity of nitrate reductase (NR, nmol/mg protein) in trunk tissues of plants growing in plots with different soil fertility levels. Bars indicate standard errors.
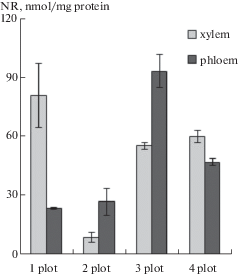
Thus, Karelian birch plants growing in plot 2 had the lowest NR activity and, therefore, the lowest assimilation of nitrates.
The activity of sucrose-metabolizing enzymes
SUS activity in the xylem of the plants exposed to low-nitrogen conditions (plot 2) and nitrogen deficit (plots 3, 4) was lower (42, 45 & 32 µmol/mg protein, respectively) than in the plants growing in plot 1 (70 µmol/mg protein). SUS activity in the phloem was 2–9 times lower than in the xylem (Fig. 4a).
Fig. 4.
The activity of sucrose synthase (SUS, µmol mg–1 protein) (a) and apoplastic invertase (ApInv, µmol/g FW) (b) in trunk tissues of plants from plots with different soil fertility levels. Bars indicate standard errors.
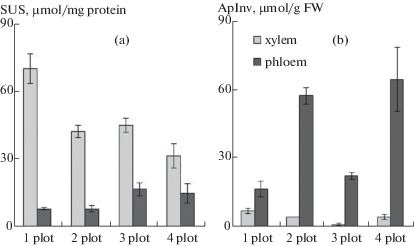
ApInv activity also varied among plots with different soil fertility levels. Its values in the phloem were 57 & 65 µmol/g FW in the plants from plots 2 & 4, and 17 & 22 µmol/g FW in the plants from plots 1 & 3. ApInv activity in the xylem was twice lower than in the phloem in the plants from plot 1, and 12–17 times lower in the plants from plots 2, 3 & 4 (Fig. 4b).
Thus, SUS activity in wood tissues was higher in the plants growing on soils with normal nitrogen availability (plot 1), whereas ApInv activity showed no correlation with natural soil fertility.
DISCUSSION
The highest expression of abnormal wood pattern (i.e. the greatest amount of dark-colored parenchymatous inclusions) combined with a high activity of ApInv in the phloem was found in the plants from plot 2, which had an intermediate level of nitrogen in the soil (Fig. 1f, j; 4b).
The plants with patterned wood getting sufficient amounts of major nutrients in plot 1 showed a 9-fold higher NR activity than the plants in plot 2 (Fig. 3), and high SUS activity as well (Fig. 4a). Increased SUS activity in plants with rich nitrate nutrition has been reported elsewhere (Nikitin, Izmailov, 2016; Galibina et al., 2016). Our previous research has shown that the increased sucrose metabolization via the SUS pathway reduces the compensatory metabolization via the invertase pathway. Then, the increased parenchymatization correlates with increased cleavage of sucrose by the invertase pathway (Galibina et al., 2015b, 2016). As a result, the plants in plot 1 have less percentage of dark parenchymatous inclusions in trunk tissues and, hence, poorer wood figure quality than the plants in plot 2.
The relationships between ApInv activity and soil conditions can be as follows. The plants in plot 2 showed a decline in SUS activity (Fig. 4a) due to a low level of soil labile nitrogen (Table) and NR activity in trunk tissues (Fig. 3). A decline in SUS activity in the xylem did not entail a decrease of tree height and diameter (Fig. 2). In patterned plants, sucrose in the differentiating xylem is actively utilized due to the activity of ApInv (Galibina et al., 2015b, 2016). This compensatory mechanism is specific to the plants with patterned wood: during active cambial growth the lower sucrose metabolization via the sucrose synthase pathway is observed alongside its increased metabolization via the invertase pathway in the xylem and, especially so, in the phloem, resulting in intensified production of hexoses in them (Galibina et al., 2015a, b; 2016; Moshchenskaya et al., 2017). Hexoses from the apoplast are delivered to the cell, where they can be utilized to replenish the UDP-glucose pool required for the synthesis of cell wall polysaccharides (Amor et al., 1992; Haigler et al., 2001; Koch, 2004; Bieniawska et al., 2007; Barratt et al., 2009; Coleman et al., 2009; Gerber et al., 2014). Thus, the patterned plants from plot 2 did not differ in height and stem diameter from the patterned plants from plot 1. On the other hand, hexoses initiate storage metabolism reactions (Koch, 2004; Smeekens, 2000; Rolland et al., 2006), which seemingly lead to a higher deposition of parenchyma in the wood of the plants from plot 2 compared to plot 1. Proceeding from the above, an assumption can be drawn that some reduction in soil fertility and assimilation of nitrates in trunk tissues is favorable for the cultivation of Karelian birch plants with rich patterned wood in tall and thick trunks.
It should be noted that the demand for nutrients in birch depends on stand age and productivity, and is constrained by soil conditions. Birch trees consume nutrients most actively until an age of 30 years, after which the consumption declines sharply. Annual loss of essential nutrients (N, P, K) from a circa 25-year-old birch stand 12 m high is 74, 8.3, 25 kg/ha (Bazilevich, Titlyanova, 2008).
If the soil nitrogen is deficient and the phosphorus level is high (as they were in plots 3 and 4), the assimilation of nitrates by NR in the trunk tissues of Karelian birch is increased. Nitrate ion itself is thought to be a signal molecule that can directly affect the expression of genes related to nitrate uptake, transport, assimilation, and the related carbon metabolism (Kaiser, Huber, 2001). Nitrate may also be one of the key metabolic factors that modulate the activities of protein kinase and protein phosphatase that act on each of the target enzymes and thereby can influence C flow between sucrose and amino acids (Kaiser, Huber, 2001). Increased NR activity in plants with patterned wood boosts the competition between two processes that require substantial amounts of photoassimilates, i.e. tree growth and formation of patterned wood through increased parenchymatization. In the first case (plot 3), patterned wood is formed in response to high SUS activity in the xylem (like in the plants from plot 2) and the phloem (twice higher than in the plants from plot 2) (Fig. 4a). Sucrose metabolization via the invertase pathway in these plants was inhibited. As a result, the plants in plot 3 matched the plants in plot 2 in height (Fig. 2), but they show poor patterning (Fig. 1g, k). In the latter case (plot 4), the pattern is formed due to high ApInv activity in the phloem (Fig. 4b). These plants were lower (Fig. 2), but with a higher expression of wood pattern (Fig. 1h, i).
Therefore, we can propose the following explanation for our results. The expression of abnormal wood pattern in Karelian birch is influenced by the availability of labile nitrogen in the soil. Where nitrogen availability is at a normal level (~80–100 kg/ha), patterned wood has normal expression (high SUS and ApInv activities in the xylem and ApInv activity in the phloem). Some reduction in soil fertility (nitrogen within ~70 kg/ha) leads to a decrease of SUS activity together with an increase of ApInv activity, and an increase in the amount of dark-colored inclusions in wood as well. The height and diameter of trees in this case remain unmodified. Where soil labile nitrogen is strongly deficient while phosphorus content is elevated, there is an increased assimilation of nitrates in trunk tissues. These changes in the nitrogen/phosphorus ratio enhance the competition for photoassimilates between the processes of patterned wood formation and plant growth. In the former process, the sucrose is metabolized via apoplastic pathway whereas in the latter one, the amounts of this sugar are used for cell wall formation (mostly in xylem). According to the data above, an increased supply of nitrate nitrogen to a plant, which entails a rise in NR activity, negatively affects the formation of patterned wood.
It is promising to study the influence of nitrogen and phosphorus assimilable compounds on the cambial zone of experimental trees directly to verify the stated provisions. We are planning to conduct such studies using the previously developed method of exogenous solutions injection into the trunk tissues o of woody plants (Novitskaya, Kushnir, 2006). This will provide data characterizing the metabolic status features and birch conducting tissues structure, formed under the influence of solutions of nitrogen and phosphorus assimilable compounds in a wide range of concentrations.
Список литературы
Amor Y., Haigler C.H., Johnson S., Wainscott M., Delmer D.P. 1992. A membrane-associated form of sucrose synthase and its potential role in synthesis of cellulose and callose in plants. – Proc. Natl. Acad. Sci. USA. 92 (20): 53–57. https://doi.org/10.1073/pnas.92.20.9353
Bakhmet O.N. 2012. Specific features of soil organic matter in forest landscapes of Karelia. – Lesovedenie. 2012. 2: 19–27 (In Russ.).
Barratt D.H.P., Derbyshire P., Findlay K., Pike M., Wellner N., Lunn J., Feil R., Simpson C., Maule A.J., Smith A.M. 2009. Normal growth of Arabidopsis requires cytosolic invertase but not sucrose synthase. – Proc. Natl. Acad. Sci. USA. 106 (31): 13124–13129. https://doi.org/10.1073/pnas.0900689106
Batasheva S.N. 2006. Nitrate ion in apoplast of plants: effect on photosynthesis and assimilate transport: Diss. … Cand. Sci. Kazan. 168 p. (In Russ.).
Bazilevich N.I., Titlyanova A.A. 2008. Biological Turnover on Five Continents: Nitrogen and Ash Elements in Natural Terrestrial Ecosystems. Novosibirsk. 380 p.
Bieniawska Z., Barratt D.H.P., Garlick A.P., Thole V., Kruger N.J., Martin C., Zrenner R., Smith A.M. 2007. Analysis of the sucrose synthase gene family in Arabidopsis. – Plant J. 49 (5): 810–828. https://doi.org/10.1111/j.1365-313X.2006.03011.x
Bruskova R.K., Nikitin A.V., Satskaya M.V., Izmailov S.F. 2009. Effect of nitrate on pea sucrose synthase – Russ. J. Plant Physiol. 56(1): 74–79. https://doi.org/10.1134/S1021443709010117
Chikov V.I., Avvakumova N.Yu., Bakirova G.G. 2003. Postphotosynthetic utilization of labeled assimilates in fiber flax. – Biology Bulletin. 30 (4): 377–382. https://doi.org/10.1023/A:1024818124498
Chikov V.I., Bakirova G.G. 2004. Role of the apoplast in the control of assimilate transport, photosynthesis, and plant productivity. – Russ. J. Plant Physiol. 51 (3): 420–431. https://doi.org/10.1023/B:RUPP.0000028691.49600.c2
Coleman H.D., Yan J., Mansfield S.D. 2009. Sucrose synthase affects carbon partitioning to increase cellulose production and altered cell wall ultrastructure. – Proc. Natl. Acad. Sci. USA. 106 (31): 13118–13123. https://doi.org/10.1073/pnas.0900188106
Evdokimov A.P. 1989. Biology and Culture of Karelian Birch. Leningrad Gos. Univ. Leningrad. 226 p. (In Russ.).
Forde B.G. 2002. Local and long-range signaling pathways regulating plant responses to nitrate. – Annu. Rev. Plant Biol. 53: 203–224. https://doi.org/10.1146/annurev.arplant.53.100301.135256
Friemann A., Lange M., Hachtel W., Brinkmann K. 1992. Induction of nitrate assimilatory enzymes in the tree Betula pendula. – Plant Physiol. 99 (3): 837–842. https://doi.org/10.1104/pp.99.3.837
Fujii S., Hayashi T., Mizuno K. 2010. Sucrose synthase is an integral component of the cellulose synthesis machinery. – Plant Cell Physiol. 51 (2): 294–301. https://doi.org/10.1093/pcp/pcp190
Galibina N.A., Moshkina E.V., Nikerova K.M., Moshchenskaya Yu.L., Znamenskii S.R. 2016. Peroxydase activity indicates veining of curly birch. – Lesovedenie. 4: 294–304 (In Russ.).
Galibina N.A., Novitskaya L.L., Krasavina M.S., Moshchenskaya Yu.L. 2015a. Activity of sucrose synthase in trunk tissues of Karelian birch during cambial growth. – Russ. J. Plant Physiol. 62 (3): 381–389. https://doi.org/10.1134/S102144371503005X
Galibina N.A., Novitskaya L.L., Krasavina M.S., Moshchenskaya Yu.L. 2015b. Invertase activity in trunk tissues of Karelian birch. – Russ. J. Plant Physiol. 62 (6): 753–760. https://doi.org/10.1134/S1021443715060060
Galibina N.A., Novitskaya L.L., Nikerova K.M. 2016. Excess of exogenous nitrates inhibits formation of abnormal wood in the Karelian birch. – Russ. Dev. Biol. 47 (2): 69–76. https://doi.org/10.1134/S106236041602003X
Gerber L., Zhang B., Roach M., Rende U., Gorzsas A., Kumar M., Burgert I., Niittylla T., Sundberg B. 2014. Deficient sucrose synthase activity in developing wood does not specifically affect cellulose biosynthesis, but causes an overall decrease in cell wall polymers. – New Phytol. 203 (4): 1220–1230. https://doi.org/10.1111/nph.12888
Gordon A.J., Minchin F.R., James C.L., Komina O. 1999. Sucrose synthase in legume nodules is essential for nitrogen fixation. – Plant Physiol. 120 (3): 867–877. https://doi.org/10.1104/pp.120.3.867
Gordon A.J., Skøt L., James C.L., Minchin F. R. 2002. Short term metabolic responses of soybean root nodules to nitrate. – J. Exp. Bot. 53 (368): 423–428. https://doi.org/10.1093/jexbot/53.368.423
Haigler C.H., Ivanova-Datcheva M., Hogan P.S., Salnikov V.V., Hwang S., Martin K., Delmer D.P. 2001. Carbon partitioning to cellulose synthesis. – Plant Mol. Biol. 47 (1): 29–51. https://doi.org/10.1023/A:1010615027986
IUSS Working Group WRB. 2015. World Reference Base for Soil Resources 2014, update 2015. International soil classification system for naming soils and creating legends for soil maps. World Soil Resources Reports 106. FAO, Rome.
Kaiser W.M., Huber S.C. 2001. Post-translational regulation of nitrate reductase: Mechanism, physiological relevance and environmental triggers. – J. Exp. Bot. 52 (363): 1981–1989. https://doi.org/10.1093/jexbot/52.363.1981
Koch K.E. 2004. Sucrose metabolism: regulatory mechanisms and pivotal roles in sugar sensing and plant development. – Curr. Opin. Plant Biol. 7 (3): 235–246. https://doi.org/10.1016/j.pbi.2004.03.014
Koch K.E., Zeng Y. 2002. Molecular approaches to altered C partitioning: genes for sucrose metabolism. – J Am. Soc. Hortic. Sci. 127 (4): 474–483.
Lyubavskaya A.Ya. 1978. Karelian Birch. Lesnaya Promyshlennost’. Moscow. 158 p. (In Russ.).
Moshchenskaya Yu.L., Galibina N.A., Topchieva L.V., Novitskaya L.L. 2017. Expression of genes encoding sucrose synthase isoforms during anomalous xylogenesis in Karelian birch. – Russ. J. Plant Physiol. 64 (4): 616–624. https://doi.org/10.1134/S1021443717030104
Nikitin A.V., Bruskova R.K., Andreeva T.M., Izmailov S.F. 2010. Effect of ammonia on sucrose synthase in pea roots Pisum sativum L. – Russ. J. Plant Physiol. 57 (1): 76–80. https://doi.org/10.1134/S1021443710010097
Nikitin A.V., Izmailov S.F. 2016. Enzymes of sucrose dissimilation as targets for nitrate in early ontogenesis of garden pea. – Russ. J. Plant Physiol. 63 (1): 152–157. https://doi.org/10.1134/S1021443715060138
Novitskaya L.L. 1998. Regeneration of bark and formation of abnormal birch wood. – Trees: Structure and Function. 13 (2): 74–79. https://doi.org/10.1007/s004680050189
Novitskaya L.L. 2008. Karelian birch: mechanisms of growth and development of structural abnormalities. Publishing House Verso. Petrozavodsk. 144 p. (In Russ.).
Novitskaya L.L., Galibina N.A., Nikerova K.M. 2015. Sugar transport and storage in the phloem of Betula pendula Roth var. pendula and var. carelica. – Transactions of the Karelian Research Centre of the Russian Academy of Sciences. Experimental biology Series. 11: 35–47. DOI: (In Russ.).https://doi.org/10.17076/eb216
Novitskaya L.L., Kushnir F.V. 2006. The role of sucrose in regulation of trunk tissue development in Betula pendula Roth. – J. Plant Growth Regul. 25 (1): 18–29. https://doi.org/10.1007/s00344-004-0419-2
Novitskaya L., Nikolaeva N., Galibina N., Tarelkina T., Semenova L. 2016. The greatest density of parenchyma inclusions in Karelian birch wood occurs at confluences of phloem flows. – Silva Fennica. 50 (3): 1461–1478. https://doi.org/10.14214/sf.1461
Rolland F., Baena-Gonzalez E., Sheen J. 2006. Sugar sensing and signaling in plants: conserved and novel mechanisms. – Annu Rev. Plant Biol. 57: 675–709. https://doi.org/10.1146/annurev.arplant.57.032905.105441
Ruan Y.L., Llewellyn D.J., Furbank R.T. 2003. Suppression of sucrose synthase gene expression represses cotton fiber cell initiation, elongation, and seed development. – The Plant Cell Online. 15 (4): 952–964. https://doi.org/10.1105/tpc.010108
Smeekens S. 2000. Sugar-induced signal transduction in plants. – Annu. Rev. Plant Physiol. Plant Mol. Biol. 51: 49–81. https://doi.org/10.1146/annurev.arplant.51.1.49
Song D., Shen J., Li L. 2010. Characterization of cellulose synthase complexes in Populus xylem differentiation. – New Phytol. 187 (3): 777–790. https://doi.org/10.1111/j.1469-8137.2010.03315.x
Sturm A., Tang G.Q. 1999. The sucrose-cleaving enzymes of plants are crucial for development, growth and carbon partitioning. – Trends Plant Sci. 4 (10): 401–407. https://doi.org/10.1016/S1360-1385(99)01470-3
Дополнительные материалы отсутствуют.
Инструменты
Ботанический журнал