Сенсорные системы, 2019, T. 33, № 2, стр. 113-123
Contribution of the marginal peripheral retina to color constancy: Evidence obtained due to contact lens with implanted occluder
G. I. Rozhkova 1, *, Е. Н. Иомдина 2, О. М. Селина 2, А. В. Белокопытов 1, П. П. Николаев 1
1 Institute for Information Transmission Problems (Kharkevich Institute), Russian Academy of Sciences
127051 Moscow, B. Karetny per. 19, Build. 1, Russia
2 Moscow Helmholtz Research Institute of Eye Diseases
105062 Moscow, Sadovaya-Chernogryazskaya, 14/19, Russia
* E-mail: gir@iitp.ru
Поступила в редакцию 15.01.2019
Аннотация
Novel experimental data are presented to demonstrate participation of the marginal peripheral retina (MPR) in providing color constancy. According to A. Yarbus (Yarbus, 1975a, b; 1977; Yarbus, Rozhkova, 1977), the task of the MPR is to estimate spectral characteristics of the scene illumination presuming that they are directly related to the ambient light stimulating MPR. The estimates of illumination obtained at the MPR are supposed to be used for color normalization by perceptual color constancy mechanisms in all the visual field. Usually, MPR photoreceptors are stimulated by two principally different light flows: the light that enters the eye through the pupil and partially scatters inside all the eye media (pupillary component) and the light that reaches the retina from its outer side, e.i. “diasclerally”, going from the illuminated eye surface through all eye tunics (scleral component). These two components differ in their spectra because they have to pass different eye structures that make the scleral component more reddish than the pupillary one. Taking into account this difference, we have contrived a new experimental paradigm for verifying Yarbus’s hypothesis on the basis of changing the balance between these two components by means of contact lens with implanted opaque occluder. The occluder diminished the amount of scattered light reaching MPR photoreceptors from inside the eye ball but did not change the outside light flow. It was anticipated that resulting change of balance in favor of reddish scleral component should lead to relative increase of the red color coordinate in the red-green-blue estimates of illumination and, correspondingly, to shifting all the perceived colors in green-blue direction. Our experimental data on peripheral vision obtained in photopic conditions of illumination supported this prediction. In certain cases, transformations of colors appeared to be dramatic, e.g. saturated red could be perceived as saturated green.
INTRODUCTION
The phenomenon of color constancy was firstly mentioned in literature long ago and was studied by many outstanding scientists: for review see (Foster, 2011). However, clarifying its physiological mechanisms is still currently one of the central problems in visual psychophysiology: for discussion see (Logvinenko et al., 2015). Despite great achievements in morphological, psychophysical and psychological studies of human visual system structure and functioning, some aspects of color constancy phenomenon are not fully understood up to now. The purpose of color constancy mechanisms is to provide invariance of perceived object coloration despite significant changes of illumination. A general basic idea underlying many theories of color vision implies normalization of local functional unit responses determined by photoreceptors selectively sensitive to long-, middle- and shortwave light (L, M, S cones): each response has to be scaled in accordance with the amount of corresponding (red, green, blue) radiation in the illumination of the observed object. The theories developed by different authors vary mainly in the suggested ways of getting the estimates of illumination spectrum. Our purpose was to validate an original hypothesis of Alfred Yarbus (Yarbus, 1975a, b) who put forward an idea that, in human eye, there is a special structure for getting the estimates of illumination spectrum – the marginal peripheral retina (MPR). According to Yarbus, MPR is a circular retinal zone running along ora serrata – the line determining anterior edge of the visual retina and its separation from the ciliary body. In Yarbus’s opinion, MPR is a blind zone that is incapable to evoke visual sensations but participates in visual process indirectly providing the estimates of illumination spectrum needed for normalization.
Actually, the idea that the extreme peripheral retina might have a direct relationship to color constancy emerged much earlier. Also, some specific experimental data on peripheral color vision alluded to such a function. However, these investigations remained incomplete and many issues remained open. Corresponding publications that appeared mostly in XX century (Hellpach, 1900; Pikler, 1931; Schouten, Ornstein, 1939; Fry, Alpern, 1953; Mollon et al., 1998; To et al., 2011; and oth.) were briefly analyzed in our previous papers (Rozhkova et al., 2016; Nikolaev, Rozhkova, 2015, 2017).
Yarbus had published his hypothesis more than fifty years ago. Since then, due to scientific progress, the notions of visual system structure, neurophysiology of vision and visual image processing underwent a great change. The number of brain areas considered as the visual ones increased more than ten times; neurophysiologic studies revealed the existence of various parallel pathways designed for the analysis of form, color, movement, etc.; psychophysical and psychological investigations demonstrated a complex heterarchy of visual modules functioning at different levels of retinal image processing. At present, it seems evident that color constancy is provided by a number of principally different mechanisms – from the sensory level of optical image transformation into neural image to the higher brain levels combining all the data of image processing in different modules and partial solutions of parallel subsystems in order to create the resulting single image and visualize it as a conscious percept. However, at the time of Yarbus’s investigations, it seemed realistic to devise relatively simple one-level theory supposedly explaining all color constancy phenomena.
In Yarbus’s theory, the perceived color of an image projected to a given retinal locus i is determined by the following three values:
(1)
${\text{ln}}({{r}_{i}}{\text{/}}{{r}_{o}});\quad {\text{ln}}({{g}_{i}}{\text{/}}{{g}_{o}});\quad {\text{ln}}({{b}_{i}}{\text{/}}{{b}_{o}}),$Yarbus thought that, in natural viewing conditions, all MPR points were always stimulated equally. However, there are reasons to suppose that it is not true. One could share Yarbus’s opinion that the ambient light scattered within the eye could be distributed over all the MPR evenly but what about the light reaching the MPR from the eye ball surface? When the subject looks not straight ahead but to the left or to the right, the MPR part under the open eye ball surface is stimulated by the light penetrating to photoreceptors through the sclera and other eye tunics while the remaining MPR part is not stimulated in a similar way since it is hidden in the eye orbit (Fig. 1, a). Moreover, the eye ball tunics are not homogeneous and, therefore, the diascleral light stimuli are different at different retinal points (Panova et al., 2018).
Fig. 1.
Scattered light flows reaching MPR photoreceptors.
a – horizontal section of the eye turned nasally showing different paths of light rays reaching the MPR at the temporal and nasal sides;
b – section of the retina showing the paths of the light rays reaching the photoreceptors through the pupil and through the eye tunics: 1 – sclera; 2 – choroid; 3 – pigment epithelium; 4 – photoreceptors; 5 – ganglion cell axons.
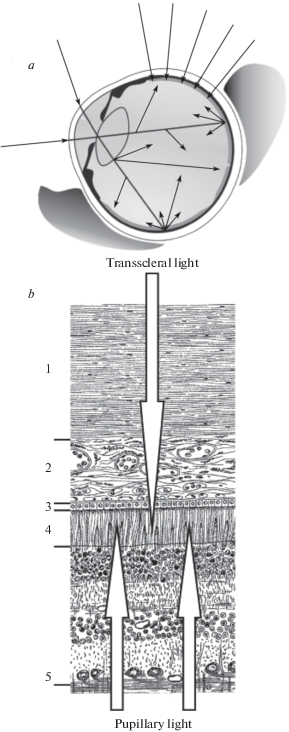
It is easy to see that, in a general case, each local retinal light stimulus consists of two or three flows. At the MPR, the triad ro, go, bo is determined by the responses to the following two possible light flows (Fig. 1): (1) the light that entered the eye through the pupil and was scattered by the internal eye structures creating almost uniform background illumination of all the retina (pupillary flow), and (2) the light that came to the receptors from the illuminated eye surface through all the eye ball tunics – conjunctiva, sclera, choroid, pigment epithelium (diascleral, or transscleral flow).
Let denote the intensities of the input light components received by L, M, S cones at the MPR through the pupil and through the eye tunics as Rop, Gop, Bop and Ros, Gos, Bos, respectively (the index p is from “pupillary” and the index s is from “scleral”). Then the responses ro, go, bo should be determined by the sums (Rop + Ros); (Gop + Gos); (Bop + Bos). At the retinal area with projected image, the triad ri, gi, bi should be determined by three light flows: the light coming from the observed object with components Rii, Gii, Bii and the background light consisting of the two light flows described above. It is evident that the pupillary background stimuli (Rop, Gop, Bop) will be present at every retinal point whereas the scleral background stimulus (Ros, Gos, Bos) should be added or not added to the projected image stimulus (Rii, Gii, Bii) depending on the image location on the retina (under illuminated open anterior eye surface or under the surface hidden in the eye orbit) as shown in Fig. 1, a.
Thus, for the retina under illuminated eye surface (far peripheral vision) one could write:
(2)
${{g}_{i}}{\text{/}}{{g}_{o}} = {\text{ }}{{g}_{i}}({{G}_{{ii}}},{{G}_{{op}}},{{G}_{{os}}}){\text{/}}{{g}_{o}}({{G}_{{op}}},{{G}_{{os}}});$(3)
${{g}_{i}}{\text{/}}{{g}_{o}} = {\text{ }}{{g}_{i}}({{G}_{{ii}}},{{G}_{{op}}}){\text{/}}{{g}_{o}}({{G}_{{op}}},{{G}_{{os}}});$In the cases corresponding to the far peripheral areas where the retinal layers spread under open eye surface, the scleral stimulus components influence both numerators and denominators (2) while in the more central retinal areas, the scleral components only influence denominators (3). It is easy to see that this difference could contribute to the character of chromaticity change with increasing eccentricity of a given test object.
Typical transformations of perceived colors commonly described in the literature on peripheral vision (Purkinje, 1823, 1825; Baird, 1905; Lythgoe, 1931; Kravkov, 1950) were recorded in natural viewing conditions with habitual ratios of the pupillary and scleral light components stimulating the MPR photoreceptors. The approach suggested by Yarbus to confirm his hypothesis about the role of the MPR was based on the idea of artificial increasing denominators in (1) by means of an additional diascleral stimulation of the MPR area. Such increase should lead to overall decrease in the perceived image brightness and, probably, to changes of the perceived colors (in the case of significant changing the relative values of ro, go, bo). In particular, the ratios between ro, go, bo could undergo changes with changing proportions between the pupillary and scleral flows having different spectra in a general case. Usually, the scleral component is more reddish than the pupillary one due to filtering of the input light in a dense net of blood vessels (choroid) on the way between sclera and retina. This means that increase of denominator should be larger for the red components than for the green and blue ones. In perceived images, such inequality would be expressed as relative suppression of red component and, therefore, as shifting the colors in green-blue direction.
In his publications, Yarbus presented some experimental evidence of anticipated transformations but his findings remained almost unknown and couldn’t be reproduced directly by other investigators because, in published descriptions of Yarbus’s experiments, some necessary technical details were omitted (Yarbus, 1975a, b; 1977). Yarbus projected local light stimuli onto the eye ball surface (in the form of narrow rings around the limbus or small spots along the horizontal meridian of the eye ball) to increase the scleral component of the background light and recorded the changes in visibility of the observed central stimuli. He wrote that, after local illumination of the eye surface, subjects could observe an overall darkening of the viewed central images as well as transformation of the perceived red test color into dark blue-green (Yarbus termed this color as “black-blue-green”) in presence of bright red scleral stimuli.
Recently, we tried to reproduce Yarbus’s results improving his experimental technique. Our setup provided a possibility to control scleral stimulus location and parameters and to obtain quantitative estimates of its influence on perception of the foveal stimuli. It was found that local stimulation of the open eye surface could make worse foveal contrast sensitivity and color recognition (Rozhkova et al., 2018). However, it appeared that foveal perception could be influenced by transscleral stimulation not only at the area of MPR but also at the retinal mid-periphery. Though stimulation of the MPR evoked significantly larger effects than stimulation of other retinal areas, no exclusive manifestation of the MPR role was observed. Thus, on one hand, we failed in obtaining direct and unambiguous evidence of Yarbus’s rightness but, on the other hand, there remained a suspicion that the effects evoked by diascleral stimulation of the retinal mid-periphery could be actually caused by a secondary increase of the MPR stimulation due to the light scattered from the area of mid-peripheral stimulus.
In this paper, we present a novel approach to validate Yarbus’s hypothesis using opaque occluder implanted in contact lens. This approach is based on our idea of changing the balance between the two components of the background light in favor of the scleral component by means of decreasing the amount of light coming to the MPR photoreceptors through the pupil. Since the diascleral light is more reddish than the pupillary light, the “red” denominator could be increased in a larger degree than “green” and “blue” ones thus leading to certain shift of the perceived colors in green-blue direction.
MATERIALS AND METHODS
To assess contribution of the MPR to color perception, systematic perimetric data on color detection and recognition were obtained for the test stimuli of various colors, intensities, and eccentricities in conditions of wearing and not wearing contact lens with implanted occluder. The occluder significantly reduced the input light flow through the cornea and the pupil shading a large part of the retina.
Experimental setup. A modified commercial perimeter PNR-2 (analogous to the Förster perimeter) was used for test stimulus presentation (Fig. 2).The arc of the perimeter was lengthened on both ends to extend the angular range of stimulation up to 125° to the left and to the right from the central point.
Fig. 2.
A modified perimeter of Förster type with elongated arcs and a movable arrangement for stimulus presentation.
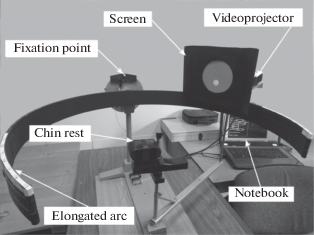
Computer-generated stimuli were projected onto a small semi-transparent screen by means of a miniature video projector (Optoma ML750ST). The screen and the apparatus for stimulus projection were placed on the platform that could be moved along the perimeter arc. Spectral characteristics of the test stimuli, corresponding to the basic R, G, B radiations of the projector are shown in Fig. 3.
Fig. 3.
Spectral characteristics of the red (a), green (b), blue (c) test stimuli (black lines) superimposed on the cone sensitivities curves (grey lines) calculated according to (Fairchild, 2007).
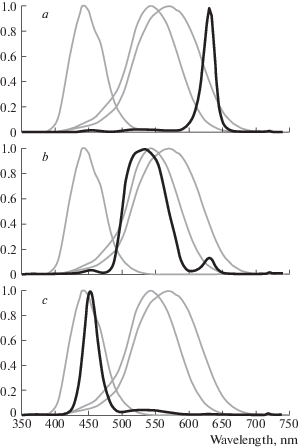
Stimuli. The parameters of the test stimuli (size, brightness, color, temporal characteristics) were controlled by means of special software. In most experiments, the test stimuli were flickering (1.5 s on and 1.5 s off) circular light spots 2 cm in diameter (angular size 4°); in some cases we also used the stimuli of 1 and 4 cm in diameter. Observation distance was 30 cm. Each test stimulus corresponded to one of the three basic colors (red, green, blue) and its physical brightness was varied in the range 1.5–970 cd/m2 using computer settings.
Color stimulus brightness was set by software in 8-bit discrete 256 RGB values and was recalculated into physical intensity in cd/m2 using the data of photometric measurements with X-Rite Eye One Pro spectrophotometer at the maximal values of RGB (255) and the native value of gamma parameter for the video projector used (2.2). Spectral characteristics of the background (“black screen”) radiance and the test stimuli (red, green and blue) are shown in Fig. 4, a, c, d. Relations between 8-bit RGB values and the data of photometric measurements are shown in Fig. 4, b.
Fig. 4.
Spectral characteristics of the stimuli (a, c, d) and relations between 8-bit RGB intensity values and the data of photometric measurements (b).
a – background radiance (R=G=B=0);
b – stimulus brightness of basic colors R, G and B in cd/m2 (y axis) for various 8-bit values (x axis);
c, d – stimulus spectra of basic colors R, G, B for 8-bit values 30 (c) and 100 (d); y axis – intensity in A.U.; x axis – wavelength in nm.
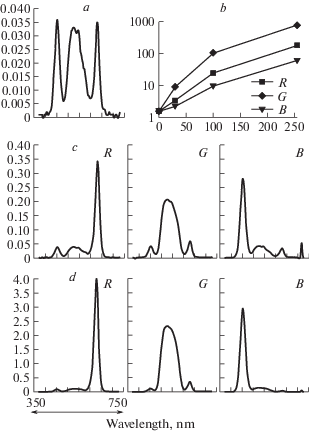
The experiments were carried out in the room with ambient illumination either 50 lx or 250 lx.
Subjects. The subjects were 7 adult volunteers aged 28–75 yr., 5 males, 2 females with good visual acuity and normal color vision (judged by optometric investigation and examination with color tests of Rabkin (1971)). In half of the experiments, the subjects wore specially designed contact lens with implanted central occluder.
Contact lens parameters. Contact lenses were of a standard universal type designed for cornea protection but not for optical correction of refractive anomalies. The implanted occluders were completely opaque. The schema of the contact lens with implanted occluder is shown in Fig. 5.
In the experiments presented in this paper, the diameter of the occluder was 8 mm. Such a size was chosen taking into account optics and geometry of the human eye anterior segment. In all cases, the pupil was completely covered with the occluder (Fig. 6). The width of the corneal peripheral ring that remained not covered was 1.5–2.0 mm that provided a possibility to observe the peripheral part of the visual field from 40–60° to its very end. The exact range depended on the individual eye features.
Fig. 6.
The photographs of the eyes with attached contact lens.
(a, b) – the same subject, contact lens 10 and 8 mm in diameter;
(b, c) – different subjects, contact lens 8 mm in diameter;
The pupil of the test eye is completely covered with occluder as can be judged by comparison with the paired eye.
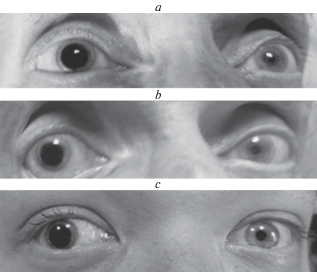
Experimental procedures. The procedure of the experiments not implying employment of contact lens didn’t differ significantly from the commonly accepted standard protocol. After positioning the testing eye at the axis of the perimeter, the subject was instructed to look monocularly, as steadily as possible, at a small red fixation point above the center of the perimetric arc. The paired eye was covered with an opaque patch. After stimulus presentation at a desired visual angle, the subject has to confirm detection of the stimulus and to describe its appearance.
At the beginning of each experiment implying employment of contact lens with occluder, it was necessary to determine the working range of visual angles for further investigation, i.e. the range of angles not precluded for stimulus presentation by the implanted occluder. To do this, we asked the subject to look at the fixation point binocularly and, using a standard procedure of stimulus presentation, determined the range of visual angles where the stimuli could be perceived with the investigated eye. At the two ends of this range (the nasal and the temporal ones), vertical bars (well seen) were fixed on the perimetric arc. During subsequent measurements, these bars served as the markers for keeping stable position of the investigated eye since the paired eye was covered with an opaque patch and couldn’t see the central fixation point and the investigated eye couldn’t see it as well because of occluder. Thus, instead of fixating invisible central point, the subject could adjust his investigated eye position properly by managing to view simultaneously the markers on both ends of his angular working range. It is noteworthy that the task of keeping the investigated eye in stable position appeared to be rather easy because, in conditions of preventing central vision, the eye practically had no tendency to perform saccades to the location of peripheral stimulus. It seemed that inability to provide foveation of the stimulus could significantly influence oculomotor behavior suppressing the saccades that became useless in such conditions.
Subject’s task was to detect stimulus appearance and to describe its perceived shape, color and contour sharpness. The experimental protocol included measuring the brightness levels for
– detecting formless flicker,
– recognizing color hue,
– perceiving saturated color,
– seeing sharp contour of the stimuli.
However, the procedure of testing was not rigorous: we tried not to influence the subject’s original spontaneous responses, on one hand, and to provide the conditions for easy comparison of the data using the same intensity levels and visual angles in different experimental sessions, on the other hand.
At the end of the experiment, taking into account the results of the threshold measurements, the stimuli with crucial parameters were slowly moved to and fro along the perimeter arc in order to verify the discovered color transformations in dynamic conditions of stimulus presentation. Since the test stimuli were flickering, the movement was not uniform but stepwise.
RESULTS AND DISCUSSION
In conditions of wearing contact lens, perimetric investigations of color perception were carried out in the ranges of the visual angles limited by the implanted occluders. The measurements were performed mostly in the temporal half of the visual field at several stimulus positions in the angular working range determined by the occluder on one of its ends and by the anatomy of the eye and the palpebral fissure on the other end.
In each subject, this working range depended on the diameter of the occluder, pupil size and the eye optics. Naturally, increase of the occluder diameter diminished the working range, however, with one and the same occluder, this range could also be essentially changed because of changing the pupil size (Fig. 7).
Fig. 7.
The schemes illustrating dependence of angular working range (or the retinal area that can be stimulated through the pupil) on the occluder diameter and the pupil size (or the level of the retina illumination):
(b) differs from (a) by larger occluder; (c) differs from (a) by larger pupil size.
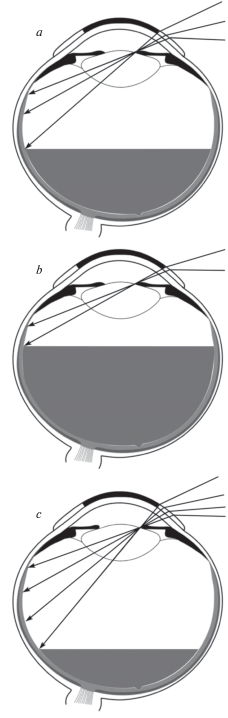
To provide comparability of the results obtained in various series of experiments and in different subjects we performed obligatory measurements at 60, 80 and 95º in the temporal part of the visual field since, in all our subjects, these angles appeared to be inside the working ranges and were suitable to observe significant color transformations.
The variety of experimental results obtained could be inferred from the data presented in the Tables 1–4.
Table 1.
The colors perceived by subject EE in conditions of wearing contact lens with 8 mm occluder when red, green and blue stimuli of various RGB values (V) were presented at the eccentricities of 60; 80 and 95°. Ambient light 250 lx
Test color | Eccentricity | |||||
---|---|---|---|---|---|---|
60° | 80° | 95° | ||||
V | Perceived image | V | Perceived image | V | Perceived image | |
R E D |
55 | Greenish flicker | 60 | Achromatic flicker | 70 | Achromatic flicker |
100 | Reddish spot | 120 | Distinct green spot | 100 | Green-blue spot | |
255 | Distinct red circle | 255 | Saturated green circle | 255 | Bright unsaturated green-blue circle | |
G R E E N |
30 | Achromatic flicker | 30 | Faintly greenish flicker | 50 | Achromatic flicker |
100 | Saturated green spot | 100 | Certainly green round spot | 100 | Green round spot | |
255 | More bright saturated green spot | 255 | Round green spot | 255 | Bright green circle | |
B L U E |
75 | Achromatic flicker | 70 | Bluish flicker | 95 | Achromatic flicker |
100 | Blue-violet spot | 100 | Violet spot | 160 | Violet flicker | |
255 | Blue-blue-violet round spot | 255 | Bright violet round spot | 255 | Bright violet round spot |
Table 2.
The colors perceived by subject EE without occluder in conditions of stimulation comparable to theTable 1
Test color | Eccentricity | |||||
---|---|---|---|---|---|---|
60° | 80° | 95° | ||||
V | Perceived image | V | Perceived imager | V | Perceived image | |
R E D |
40 | Reddish flicker | 60 | Achromatic flicker | 90 | Achromatic flicker |
100 | Bright red spot like red LED | 100 | Distinct green | 170 | Greenish spot | |
255 | More bright and saturated red spot | 255 | Light green | 255 | More bright greenish spot | |
G R E E N |
15 | Achromatic flicker | 30 | Achromatic flicker | 40 | Achromatic flicker |
50 | Distinct green spot | |||||
100 | Bright light green | 110 | Distinct green spot | 100 | Good green spot | |
255 | More bright and saturated green | 255 | Saturated emerald ellipse | 255 | Bright light green spot | |
B L U E |
40 | Flicker with blue hue | 60 | Achromatic flicker with blue hue | 110 | Achromatic flicker |
100 | Blue-violet spot | 100 | Dark blue-violet spot | 150 | Flicker with light green hue | |
255 | Bright blue-blue-violet spot | 255 | Dark blue spot | 255 | Light blue spot |
Table 3.
The colors perceived by subject EE without occluder in conditions of stimulation comparable to Table 2 but with ambient light 50 lx
Test color | Eccentricity | |||||
---|---|---|---|---|---|---|
60° | 80° | 95° | ||||
V | Perceived image | V | Perceived image | V | Perceived image | |
R E D |
15–20 | Achromatic flicker with reddish hue | 25–35 | Achromatic flicker with reddish hue | 40 | Achromatic flicker |
100 | Distinct red spot | 100 | Distinct red spot | 100 | Greenish spot | |
255 | Bright red spot | 255 | Saturated blood-red ellipse | 255 | Less saturated greenish spot | |
G R E E N |
10 | Greenish flicker | 10 | Greenish flicker | 25 | Achromatic flicker |
100 | “Laser” green spot | 100 | Distinct green spot | 100 | Distinct green spot | |
255 | More bright green spot | 255 | More saturated green spot | 255 | Bright unsaturated green spot | |
B L U E |
15 | Achromatic-bluish flicker | 25 | Bluish flicker | 40 | Achromatic-bluish flicker |
100 | Distinct blue spot | 100 | Blue-violet spot | 100 | Dark blue spot | |
255 | Bright light blue spot | 255 | Bright blue spot | 255 | Bright light blue spot |
Table 4.
Inter-individual variability of color transformations observed under changes of the red test stimulus eccentricity and intensity. Data of 3 subjects wearing contact lens with occluder 8 mm. Ambient light level 250 lx
Subject | Eccentricity | |||||
---|---|---|---|---|---|---|
60° | 80° | 95° | ||||
V | Perceived image | V | Perceived image | V | Perceived image | |
NV | 55 | Flicker with slight orange hue | 60 | Achromatic flicker | 80 | Achromatic flicker |
80 160 | Orange-red spot (like unripe, then more ripe tomato) | 120 I50 | Coral hue appears and became distinct | 130 | Yellowish object like incandescent lamp | |
255 | Bright red spot (of red flag color) | 255 | Saturated coral spot | 255 | Yellowish spot | |
EE | 55 | Greenish flicker | 60 | Achromatic flicker | 70 | Achromatic flicker |
100 | Reddish spot | 120 | Distinct green spot | 100 | Green-blue spot | |
255 | Distinct red circle | 255 | Saturated green circle | 255 | Bright unsaturated green-blue circle | |
IN | 60 | Reddish flicker | 50 | Achromatic flicker | 60 | Achromatic flicker |
100 | Distinct red spot | 100 | Red spot | 100 | Distinct red flicker | |
255 | Bright saturated red spot (of red flag color) | 255 | Very bright red spot | 255 | Distinct red spot |
Tables 1 and 2 show brief protocols of one and the same subject EE examined with and without occluder on his testing eye in identical experimental conditions. Comparing these tables, one could assess the effect of occluder. It is easy to see that, in the data of Table 1 corresponding to the case of wearing contact lens with occluder, a tendency to perceive green and green-blue colors in the cases of red test stimuli is more strongly expressed than in the Table 2.
Table 3 shows similar protocol of the same subject EE examined without occluder in conditions of stimulation comparable to Table 2 but with ambient light level lowered from 250 to 50 lx. Comparing the Tables 2 and 3, one could assess the effect of the ambient light level. It is easy to notice that decrease of the ambient light significantly diminished all flicker thresholds and made the perceived colors of the test stimuli closer to the “true” hue: e.g. blue test became seen as blue but not violet (see the last lines corresponding to the intensity level 255 in the two Tables). One could suggest three reasons, at least, for such changes in the case of lower illumination level: (1) the pupil size became larger leading to increase of the stimulus image physical intensity on the retina, (2) at the MPR, the balance between the pupillary and the scleral components of the scattered light changed in favor of the pupillary component, i.e. became “more natural”, and (3) sensitivity of the retina became higher due to neurophysiologic mechanisms of adaptation.
In accordance with our predictions, most noticeable color transformations were observed in the cases of the red test stimuli. For this reason, in Table 4, we only presented the data for such stimuli. This table illustrates inter-individual variability of color transformations observed under changes of the red test stimulus eccentricity and intensity. The presented data of 3 subjects wearing the same contact lens with occluder of 8 mm diameter were obtained in condition of the ambient light level 250 lx. The differences between individuals are evident and impressive. In conditions of increasing the red test stimulus eccentricity, the subject NV demonstrated typical transformations of the perceived color from red to yellow, the subject IN continued to perceive the stimulus as red up to 95°, and the subject EE reported seeing red as green already at 80°.
It should be noted that, increasing test stimulus intensity and/or size, we succeeded in evoking adequate hue perception in all subjects up to the eccentricity of 95°. For technical reasons, it was difficult to extend observations to the very end of the visual field in each subject. However, for peripheral vision, color perception at 95° seems to be quite good finding. In most cases, the stimulus of diameter 2 cm was sufficient for obtaining perception of saturated colors in the whole range of visual angles used. The exception was the case of the oldest subject who required twice larger stimulus diameter (4 cm) for perceiving red color at the periphery of 95°.
The anticipated color transformations were not equally well manifested in all subjects. In our experiments, we were able to confirm certain prediction but only in some subjects and in certain stimulus conditions.
The results presented in the Tables 1–4 and the analysis of concurrent observations could be briefly summarized as follows.
(1) It has been found that vivid red, green, and blue colors can be perceived at the periphery of the visual field as far as 95°, at least. These finding extends up to the extreme periphery the data of С.W. Tyler which recently created a demo to support the opinion of rather good color vision at the periphery up to 50° for sufficiently large and bright stimuli (Tyler, 2015, 2016).
(2) When the stimuli with constant parameters were slowly moved along the perimetric arc from mid to far periphery, the subjects could observe systematic transformations of perceived colors analogous to those commonly described in literature but not in all respects.
It is interesting to compare our data with a description of color changes given in the paper of Lythgoe (1931):
– all workers agree, in general, on the following changes in hue when the stimulus is viewed with increasing obliquity:
– red soon becomes orange, then yellow, yellow-brown and finally black (or grey if viewed on black background);
– orange passes through yellow to grey;
– green passes through yellow to grey;
– purple becomes violet-blue and finally grey;
– violet passes through blue to black.”
Despite overall similarity between our data and the transformations described, one could notice essential difference: Lythgoe had not mentioned a possibility of red color transformation into saturated green observed in our experimental conditions and in some experiments of Yarbus. Quantitatively, such unusual transformation could be explained by significant artificial changes of the balance between the pupillary and scleral light flows leading to corresponding changes of the color normalization process. As mentioned earlier, the diascleral light is more reddish than the internal scattered light and “red” normalizing denominator could be increased in a larger degree than “green” and “blue” ones thus leading (in certain conditions) to surprising transformation of perceive red color to green color.
Unfortunately, it is not easy to investigate the spectra of the background light flows reaching photoreceptors both through the pupil and through sclera since it requires performing measurements of light intensities inside the eye. For this reason, reliable and detailed information on this issue is absent. Spectral composition of the pupillary component depends on the optical transmission properties of the ocular media comprising cornea, aqueous and vitreous humours, and crystalline lens. On its passage through the eye, the incident light beam undergoes absorption and scatter. According to (Boettner, 1962; Ruddock, 1972), the wavelength dependence of the light absorption in the eye is almost completely due to crystalline lens, whereas the cornea, aqueous and vitreous humours are transparent in the visible range of the optical spectrum. Generally, crystalline lens absorbs light in the blue part of the spectrum greater than in the red part, but degree of absorption varies significantly between subjects (Artigas, 2012). The scattering in the ocular media is also greater in the blue region of the spectrum but has weak dependence on the wavelength.
Spectral composition of the scleral component is due mainly to choroid transmission (Hammer, 1995) which is noticeably greater in the red region of the spectrum. The sclera itself and the outer tunics (conjunctiva, fascia Tenoni, pigment epithelium) exert little influence on the spectrum of the diascleral light. There are indications that inter-individual variability in light transmission along this way is also significant. It’s noteworthy that, long ago, describing his experiments with diascleral observation of the small bright images projected onto the retina through the pupil, Donders (1877) had mentioned that it was easier to perform investigations on the subjects with light eyes, probably due to some favorable peculiarities of their pigment system. In our experiments the difference between the subjects with light and dark eyes was also noticeable. It is natural to think that other individual features could influence our results as well. Thus, it is not strange that the anticipated color transformations were not equally well manifested in all subjects.
Completing this paper, it seems aptly to mention that, in human, the open area of the eye surface around the iris has the largest size among all mammals (Kobayashi, Kohshima, 2001). The adaptive meaning of this morphological property is thought to be linked to social life style and communication. However, in most cases, the existence of some outstanding property manifests itself more than in one way. Thus, it is natural to suppose that the open eye surface plays also some specific role in neural visual processing. The cause to think so is provoked by information about blindness of the peripheral retina situated under the open eye surface.
What advantages one could imagine for estimating ambient illumination by means of the blind retina responses to scattered light? Perhaps, the following consideration could be useful. The spectrum of the light reaching photoreceptors through the eye surface can be assumed as equal to S(λ)F(λ) where S(λ) – the illumination spectrum, and F(λ) – the transmission function of the eye tunics. Since F(λ) is practically independent of viewing conditions, it seems possible to get a plausible estimation of S(λ) by means of proper operations on the basis of visual experience. At the same time, the spectrum of the diffused light reaching photoreceptors through the pupil depends on the visual scene content and can be changed significantly with changing gaze direction.
Summarizing our results and sparse data available in literature one can conclude that the task of clarifying the physiological mechanisms of color constancy still need a lot of various investigations.
Some results of this work were presented at the 41st ECVP (2018, Triest, Italy).
Список литературы
Artigas J.M., Felipe A., Navea A., Fandiño A., Artigas C. Spectral transmission of the human crystalline lens in adult and elderly persons: color and total transmission of visible light. Invest Ophthalmol & Vis Sci. 2012. V. 53 (7). P. 4076–84. https://doi.org/10.1167/iovs.12-9471
Baird J.W. The color sensitivity of the peripheral retina. Washington: Carnegie Institute. 1905. No. 29.
Boettner E.A., Wolter J.R. Transmission of the Ocular Media. Invest. Ophthalmol. & Vis. Sci. 1962. V. 1 (6). P. 776–783.
Donders F.C. Die Grenzen des Gesichtsfeldes in Beziehung zudenen der Netzhaut / Albrecht. Graef’s Arch Ophthal. 1877. V. 23. P. 255–280.
Fairchild M. CIE 170-1:2006 Cone Fundamentals for Various Field Sizes and Observer Ages. Last Revision: 02/06/2007. URL: https://www.rit.edu/science/pocs/ useful-data (accessed 15.01.2019).
Foster D.H. Color constancy. Vision Research. 2011. V. 51. P. 674–700. https://doi.org/10.1016/j.visres.2010.09.006
Fry G.A., Alpern M. The effect on foveal vision produced by a spot of light on the sclera near the margin of the retina. JOSA. 1953. V. 43 (3). P. 187–188.
Hammer M., Roggan A., Schweitzer D., and G Muller. Optical properties of ocular fundus tissues-an in vitro study using the double-integrating-sphere technique and inverse Monte Carlo simulation. Physics in Medicine and Biology. 1995. V. 40 (6). P. 963–978. https://doi.org/10.1088/0031-9155/40/6/001
Hellpach W. Die Farbenwahrnehmung im indirecten Sehen. Philosophische Studien. 1900. V. 15. P. 524–578.
Kobayashi H., Kohshima S. Unique morphology of the human eye and its adaptive meaning: comparative studies on external morphology of the primate eye. Journal of Human Evolution. 2001. V. 40. P. 419–435. https://doi.org/10.1006/jhev.2001.0468
Kravkov S.V. Glaz i ego rabota: psihofiziologija zrenija, gigiena osveshhenija [The Eye and its functions: psychophysiology of vision, hygiene of illumination]. Moskva-Leningrad. Izd-vo Akademii nauk SSSR [Moscow-Leningrad. Publishing House of the Academy of Sciences of the USSR]. 1950. 531 p (in Russian).
Lythgoe R. Dark-adaptation and the peripheral colour sensations of normal subjects. Brit. J. Ophthalmol. 1931, April. P. 193–210.
Logvinenko A.D., Funt B., Mirzaei H., Tokunaga R. Rethinking colour constancy. PLOS ONE. 2015. V. 10 (9). P. e0135029. https://doi.org/10.1371/journal.pone.0135029
Mollon J., Regan B.C., Bowmaker J.K. What is the function of the cone-rich rim of the retina? Eye. 1998. V. 12 (Pt 3b). P. 548–552.
Nikolaev P.P., Rozhkova G.I. Analiz koncepcii A.L. Yarbusa o roli slepoj setchatki v cvetovosprijatii [Analysis of the Yarbus’s conceptions on the role of the blind retina in color perception]. Sensornye sistemy [Sensory systems]. 2017. V. 31 (2). P. 116–138 (in Russian).
Nikolaev P., Rozhkova G. Yarbus’s Conceptions on the General Mechanisms of Color Perception. Perception. 2015. V. 44 (8–9). P. 952–972.
Panova I.G., Poltavtseva R.A., Rozhkova G.I. Morfologicheskaya kharakteristika razvitiya krainei periferii setchatki v oblasti ora serrata [Characteristics of morphological development of the extreme retinal periphery near ora serrata]. Sensornye sistemy [Sensory systems]. 2018. V. 32 (4). P. 302–309 (in Russian). https://doi.org/10.1134/S0235009218040091
Pikler J. Das Augenhtillenlicht als Mass der Farben. Zeits. f. Psychol. 1931. B. 120 (189).
Purkinje J. Commentatio de examine physiologique organi visus et systematis cutanei. Breslau: University of Breslau. 1823.
Purkinje J. Beobachtungen und Versuche zur Physiologie der Sinne. Zweites Bändchem. In: Neue Beträge zur Kenntnis des Sehens in Subjektiver Hinsicht. Berlin. Reimer. 1825.
Rabkin E.B. Polihromaticheskie tablicy dlja issledovanija cvetooshhushhenija [Polychromatic tables for color perception test]. Moskva. Medicina [Moscow. Medicine], 1971. 72 p. (in Russian).
Rozhkova G.I., Rychkova S.I., Gracheva M.A., Belokopytov A.V., Iomdina E.N. Vliyanie lokalnoi diaskleralnoi stimulyatsii krainei i srednei periferii setchatki na fovealnuyu kontrastnuyu chuvstvitelnost i tsvetorazlichenie [The effect of local diascleral stimulation of the extreme and middle peripheral parts of the retina on foveal contrast sensitivity and color recognition]. Sensornye sistemy [Sensory systems] 2018. V. 32 (4). P. 310–320 (in Russian). https://doi.org/10.1134/S0235009218040108
Rozhkova G.I., Belokopytov A.V., Gracheva M.A. Zagadki slepoi zony i koltsa povyshennoi plotnosti kolbochek na krainei periferii setchatki [Mysteries of the blind zone and cone-enriched rim at the extreme periphery of the human retina]. Sensornye sistemy [Sensory systems]. 2016. V. 30 (4). P. 263–281 (in Russian).
Ruddock K.H. Light transmission through the ocular media and macular pigment and its significance for psychophysical investigation. In: Jameson D., Hurvich L.M. (eds) Visual Psychophysics. Handbook of Sensory Physiology, vol 7/4. 1972. Springer, Berlin, Heidelberg.
Schouten J.F., Ornstein L.S. Measurements on direct and indirect adaptation by means of a binocular method. JOSA. 1939. V. 29. P. 168–182.
To M.P.S., Regan B.C., Wood D., Mollon J.D. Vision out of the corner of the eye. Vision Research. 2011. V. 51 (1). P. 203–214.
Tyler C.W. Peripheral color demo. I-Perception. 2015. V. 6 (6). P. 2041669515613671. https://doi.org/10.1177/2041669515613671
Tyler C.W. Peripheral color vision and motion processing. Human Vision and Electronic Imaging. 2016. V. 5. P. 1–5. https://doi.org/10.2352/ISSN.2470-1173.2016.16HVEI-138
Yarbus A.L. O rabote zritel’noj sistemy cheloveka. I. Adekvatnyj zritel’nyj stimul [Human visual system. I. Adequate visual stimulus]. Biofizika [Biophysics]. 1975a. V. 20 (5). P. 916–919 (in Russian).
Yarbus A.L. O rabote zritel’noj sistemy cheloveka. II. Cvet [Human visual system. II. The perceived colour]. Biofizika [Biophysics]. 1975 b. V. 20 (6). P. 1099–1104 (in Russian).
Yarbus A.L. O rabote zritel’noj sistemy cheloveka. VIII. Opisanie operacij s cvetami sredstvami vektornoj algebry [Human visual system. VIII. Description of colour transformations by means of vector algebra]. Biofizika [Biophysics]. 1977. V. 22 (6). P. 1087–1094 (in Russian).
Yarbus A.L., Rozhkova G.I. Osobennosti vosprijatija ob#ektov na periferii polja zrenija [The peculiarities of perceiving visual objects at the periphery of the visual field]. In: Sensornye sistemy [Sensory systems]. Leningrad: Nauka. 1977. P. 64–73. (in Russian).
Дополнительные материалы отсутствуют.
Инструменты
Сенсорные системы